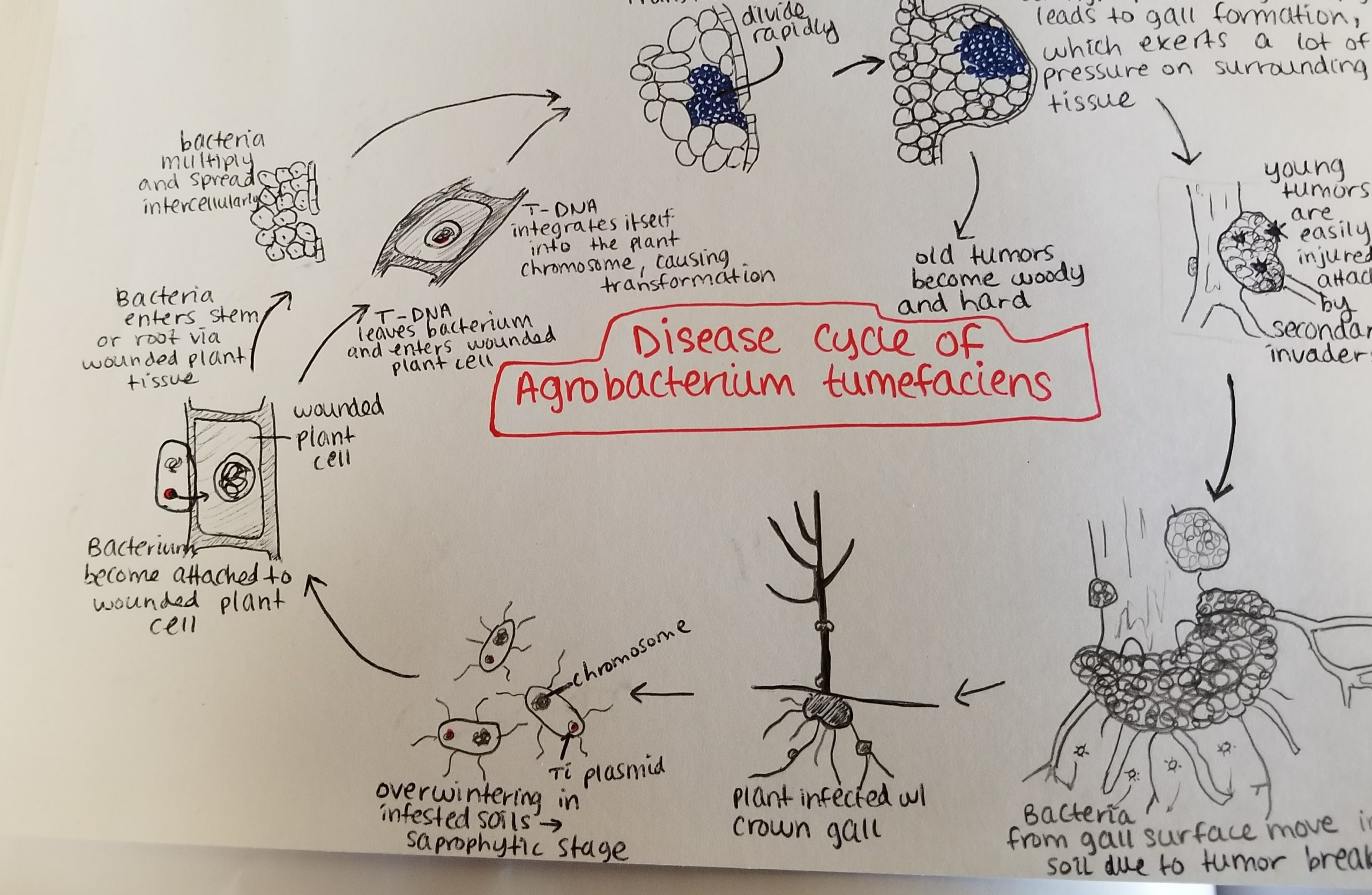
Bacterial diseases of hazelnut (Corylus avellana & Corylus spp.) and more
Bacterial blight
Xanthomonas arboricola | |
---|---|
Scientific classification | |
Domain: | Bacteria |
Phylum: | Pseudomonadota |
Class: | Gammaproteobacteria |
Order: | Xanthomonadales |
Family: | Xanthomonadaceae |
Genus: | Xanthomonas |
Species: | X. arboricola |
Binomial name | |
Xanthomonas arboricola Vauterin et al. 1995 | |
Type strain | |
ATCC 49083 |
Xanthomonas arboricola pv. corylina
Xanthomonas arboricola is a species of bacteria. This phytopathogenic bacterium can cause disease in trees like Prunus, hazelnut and walnut.
- Garrity, George M.; Brenner, Don J.; Krieg, Noel R.; Staley, James T. (eds.) (2005). Bergey’s Manual of Systematic Bacteriology, Volume Two: The Proteobacteria, Part C: The Alpha-, Beta-, Delta-, and Epsilonproteobacteria. New York, New York: Springer. ISBN 978-0-387-24145-6.
Hosts and symptoms
Xanthomonas arboricola has a vast host range, however, most symptoms are consistent throughout each of the cultivars such as hazelnut, walnut, and genus prunus (stone fruits). In X. pruni (syn. X. arboricola pv. pruni), the symptoms first usually begin with dark lesions forming on leaves. As the bacteria proliferate, bacterial ooze is noticeable as the necrotic lesions can become greasy. The discharge from the leaf can then spread inoculum to other leaves via rain splash. Infection can lead to an early defoliation of the plant, greatly affecting fruit production. Fruit will also show lesions similar to those found on the leaves. The lesions can cause significant rot of the fruit, thus decreasing or even eliminating any yield. While these are the primary symptoms, sometimes the bacteria can enter the twig over winter, and cause noticeable cankers in the spring; a dieback can happen if the stem cankers are severe enough.
- “Plantwise Technical Factsheet: walnut blight (Xanthomonas arboricola pv. juglandis)”. www.plantwise.org. Retrieved 2018-12-12.
- Boudon, Slyvain, et al. “Structure and Origin of Xanthomonas Arboricola Pv. Pruni Populations Causing Bacterial Spot of Stone Fruit Trees in Western Europe.” Phytopathology, 2005, https://apsjournals.apsnet.org/doi/pdf/10.1094/PHYTO-95-1081.
- Elphinstone, J G, and A Aspin. “Bacterial Spot and Canker of Prunus Xanthomonas arboricola pv. Pruni.” Plant Health Portal, 2016, https://planthealthportal.defra.gov.uk/assets/factsheets/x-arboricola-pv-pruni-factsheet.pdf.
Disease cycle
Xanthomonas arboricola can infect all green tissue of the plant. The disease cycle of Xanthomonas arboricola begins on the leaves of the infected plant where the bacteria will live in an epiphytic stage (gathering all nutrients and water from the air) until mid to late spring when sufficient rainsplash spreads the bacteria to new buds and fruits where it becomes pathogenic. With enough early spring rain, a repeating cycle can form in which infected leaves and young buds pass the disease to early season fruit and nutlets. With less rain, a late season infection of fruit and nuts will occur. After the infection of fruit and nuts in autumn, Xanthomonas arboricola will spread to dormant buds and wounds in leaves or branches and overwinter. Come early spring the Xanthomonas will once again enter an epiphytic stage on leaves emerging from previously infected dormant buds and repeat the cycle annually.
- Walnut Blight Management”. www.sacvalleyorchards.com. Retrieved 2017-12-15.
- Lamichhane, Jay Ram (2014). “Xanthomonas arboricola Diseases of Stone Fruit, Almond, and Walnut Trees: Progress Toward Understanding and Management”. Plant Disease. 98 (12): 1600–1610. doi:10.1094/pdis-08-14-0831-fe. PMID 30703892.
Environment impact on disease cycle
Incidence of infection can be correlated with rainfall events during the budding season of the walnut. The most important rains occurring during bud break events. This is due to the rainsplash moving inoculum from infected buds across other green tissues of the tree, most crucially to developing nuts.
- Lindow, Steven; Olson, William; Buchner, Richard (2014). “Colonization of Dormant Walnut Buds by Xanthomonas arboricola pv. juglandis Is Predictive of Subsequent Disease”. Phytopathology. 104 (11): 1163–1174. doi:10.1094/phyto-01-14-0001-r. PMID 25338268.
Importance
Xanthomonas arboricola has an extraordinarily destructive potential when infecting crops. It is the most devastating blight on walnut, and can cause up to 100% yield loss if not properly managed. Its host range threatens stone fruits and other nuts. Epidemics have been reported in countries such as The United States, Iran, Turkey, and Italy. These outbreaks are not limited to just these countries, and without extensive epidemiological knowledge, it could spread with devastating effects. Through disease forecasting efforts, X. arboricola is known to have its highest virulence and growth rate at around 30 degrees Celsius. Temperature in addition to understanding regional humidity, could help prevent future epidemics in agriculture. Less moisture on the plants, and slightly cooler temperatures can hinder both the dispersal and growth of X. arboricola. In Australia an estimated 3.1 million AUD were lost on average due to yield losses from prunus spp. in years with pathogen prevalence. The pathogen’s capability to survive in woody tissue over winter, or after plant death proves a major challenge for plant pathologists to find an effective solution.[citation needed]
- Boudon, Slyvain, et al. “Structure and Origin of Xanthomonas Arboricola Pv. Pruni Populations Causing Bacterial Spot of Stone Fruit Trees in Western Europe.” Phytopathology, 2005, https://apsjournals.apsnet.org/doi/pdf/10.1094/PHYTO-95-1081.
- Lamichhane, Jay Ram (2014). “Xanthomonas arboricola Diseases of Stone Fruit, Almond, and Walnut Trees: Progress Toward Understanding and Management”. Plant Disease. 98 (12): 1600–1610. doi:10.1094/pdis-08-14-0831-fe. PMID 30703892.
- Morales, Gerard, et al. “A Model for Predicting Xanthomonas Arboricola Pv. Pruni Growth as a Function of Temperature.” PLOS ONE, Public Library of Science, 2017, https://journals.plos.org/plosone/article?id=10.1371/journal.pone.0177583.
Management
Current management of bacterial walnut blight caused by Xanthomonas arboricola pv. juglandis is through copper-based bactericide sprays. Spraying is started just prior to early shoot emergence and continued at 7- to 10-day or longer intervals as necessary for disease control according to spring rainfall. This spraying regime is to continue until the end of August. Unfortunately, continuous use of copper-based bactericides lead to a buildup of copper in soil and can be related to yield loss in walnut orchards. A natural antibiotic produced by a strain of Streptomyces by the name of kasumin or kasugamycin has been found to have high efficacy against Xanthomonas arboricola pv. juglandis as a copper spray alternative under dry conditions. However, this same efficacy has not been found to hold up under moderate to heavy rainfall unless paired with a copper-based spray treatment.
- “UC IPM: UC Management Guidelines for Walnut Blight on Walnut”. ipm.ucanr.edu. Retrieved 2017-12-15.
- Ninot, Antònia; Aletà, Neus; Moragrega, Concepció; Montesinos, Emilio (2002). “Evaluation of a Reduced Copper Spraying Program to Control Bacterial Blight of Walnut”. Plant Disease. 86 (6): 583–587. doi:10.1094/pdis.2002.86.6.583. PMID 30823227.
- Buchner, R. (2013). “Walnut blight management using Xanthomonas arboricola pv. juglandis dormant bud population sampling” (PDF). Retrieved November 27, 2017.
With the overall lack of resistance and limited control measures, sanitation and irrigation are one of the most widely recommended modes of management. Proper irrigation and water management can help limit the amount of rain splash to prevent the rapid spread of disease. In preventing epidemics, it is recommended to plant certified propagation material; this inhibits the spread of epiphytic X. arboricola. Sanitation is crucial in stopping the distribution of this disease, given how widespread and destructive it can be.
- Lamichhane, Jay Ram (2014). “Xanthomonas arboricola Diseases of Stone Fruit, Almond, and Walnut Trees: Progress Toward Understanding and Management”. Plant Disease. 98 (12): 1600–1610. doi:10.1094/pdis-08-14-0831-fe. PMID 30703892.
- Loreti, S.; Pucci, N.; Perez, G.; Catara, V.; Scortichini, M.; Bella, P.; Ferrante, P.; Giovanardi, D.; Stefani, E. (2015). “Detection and identification of Xanthomonas arboricola pv. Pruni from symptomless plant material: Results of an Italian test performance study”. EPPO Bulletin. 45: 41–51. doi:10.1111/epp.12194. hdl:11380/1077606.
Active projects are underway to breed for resistance for Xanthomonas arboricola, however, it is difficult to implement resistant varieties of the susceptible hosts. Current efforts to breed resistance have yet to be fully successful. A study in the United States developed partial resistance; all resistance genotypes continued to show at least one symptom. One of the best modes of controlling the spread of the disease is by testing nursery plants for the bacteria before planting. This helps to guarantee that quarantined fields are not introduced to the bacteria by a foreign contaminate. Providing healthy planting material can help stop the relative spread of Xanthomonas arboricola, however, this is good practice and not a definitive solution.[citation needed]
- Elphinstone, J G, and A Aspin. “Bacterial Spot and Canker of Prunus Xanthomonas arboricola pv. Pruni.” Plant Health Portal, 2016, https://planthealthportal.defra.gov.uk/assets/factsheets/x-arboricola-pv-pruni-factsheet.pdf.
External links
- Xanthomonas LPSN J.P. Euzéby: List of Prokaryotic names with Standing in Nomenclature
- Type strain of Xanthomonas arboricola at BacDive – the Bacterial Diversity Metadatabase
- Xanthomonadales
- Bacterial tree pathogens and diseases
- Stone fruit tree diseases
- Hazelnut tree diseases
- Nut tree diseases
Bacterial canker
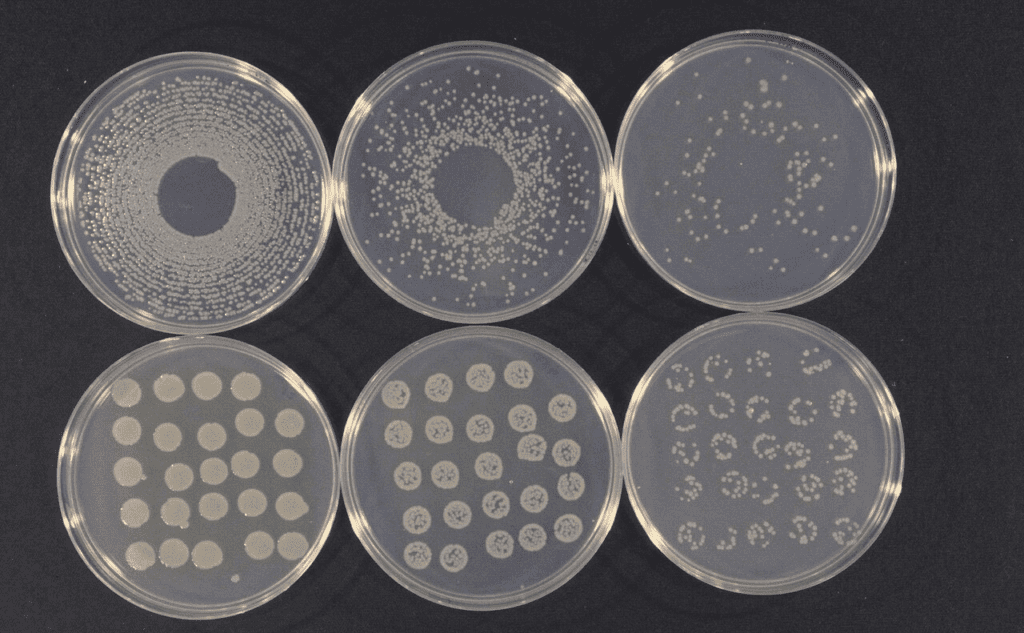
Pseudomonas syringae pv avellanae
Pseudomonas syringae is a rod-shaped, Gram-negative bacterium with polar flagella. As a plant pathogen, it can infect a wide range of species, and exists as over 50 different pathovars, all of which are available to researchers from international culture collections such as the NCPPB, ICMP, and others.
- “Pseudomonas syringae pv. persicae (PSDMPE)[Overview]”. Global Database. EPPO (European and Mediterranean Plant Protection Organization). 28 January 2001. Retrieved 22 March 2022.
- Arnold, DL; Preston, GM (2019). “Pseudomonas syringae: enterprising epiphyte and stealthy parasite”. Microbiology. 165 (3): 251–53. doi:10.1099/mic.0.000715. PMID 30427303.
Pseudomonas syringae | |
---|---|
Cultures of Pseudomonas syringae | |
Scientific classification | |
Domain: | Bacteria |
Phylum: | Pseudomonadota |
Class: | Gammaproteobacteria |
Order: | Pseudomonadales |
Family: | Pseudomonadaceae |
Genus: | Pseudomonas |
Species: | P. syringae |
Binomial name | |
Pseudomonas syringae Van Hall, 1904 | |
Type strain | |
ATCC 19310 CCUG 14279 CFBP 1392 CIP 106698 ICMP 3023 LMG 1247 NCAIM B.01398 NCPPB 281 NRRL B-1631 | |
Pathovars | |
P. s. pv. aceris P. s. pv. aptata P. s. pv. atrofaciens P. s. pv. dysoxylis P. s. pv. japonica P. s. pv. lapsa P. s. pv. panici P. s. pv. papulans P. s. pv. persicae (Prunier, Luisetti &. Gardan) Young, Dye & Wilkie P. s. pv. pisi P. s. pv. syringae P. s. pv. morsprunorum |
Pseudomonas syringae is a member of the genus Pseudomonas, and based on 16S rRNA analysis, it has been placed in the P. syringae group. It is named after the lilac tree (Syringa vulgaris), from which it was first isolated.
- Anzai, Y; Kim, H; Park, JY; Wakabayashi, H; Oyaizu, H (2000). “Phylogenetic affiliation of the pseudomonads based on 16S rRNA sequence”. International Journal of Systematic and Evolutionary Microbiology. 50 (4): 1563–89. doi:10.1099/00207713-50-4-1563. PMID 10939664.
- Kreig, N. R.; Holt, J. G., eds. (1984). Bergey’s Manual of Systematic Biology. Baltimore: Williams and Wilkins. pp. 141–99.
A phylogenomic analysis of 494 complete genomes from the entire Pseudomonas genus showed that P. syringae does not form a monophyletic species in the strict sense, but a wider evolutionary group that also included other species as well, such as P. avellanae, P. savastanoi, P. amygdali, and P. cerasi.
- Nikolaidis, Marios; Mossialos, Dimitris; Oliver, Stephen G.; Amoutzias, Grigorios D. (August 2020). “Comparative Analysis of the Core Proteomes among the Pseudomonas Major Evolutionary Groups Reveals Species-Specific Adaptations for Pseudomonas aeruginosa and Pseudomonas chlororaphis”. Diversity. 12 (8): 289. doi:10.3390/d12080289.
Pseudomonas syringae tests negative for arginine dihydrolase and oxidase activity, and forms the polymer levan on sucrose nutrient agar. Many, but not all, strains secrete the lipodepsinonapeptide plant toxin syringomycin, and it owes its yellow fluorescent appearance when cultured in vitro on King’s B medium to production of the siderophore pyoverdin.
- Scholz-Schroeder, Brenda K.; Soule, Jonathan D.; Gross, Dennis C. (2003). “The sypA, sypB, and sypC Synthetase Genes Encode Twenty-Two Modules Involved in the Nonribosomal Peptide Synthesis of Syringopeptin by Pseudomonas syringae pv. syringae B301D”. Molecular Plant-Microbe Interactions. 16 (4): 271–80. doi:10.1094/MPMI.2003.16.4.271. PMID 12744455.
- Cody, YS; Gross, DC (1987). “Characterization of Pyoverdin(pss), the Fluorescent Siderophore Produced by Pseudomonas syringae pv. syringae”. Applied and Environmental Microbiology. 53 (5): 928–34. Bibcode:1987ApEnM..53..928C. doi:10.1128/AEM.53.5.928-934.1987. PMC 203788. PMID 16347352.
Pseudomonas syringae also produces ice nucleation active (INA) proteins which cause water (in plants) to freeze at fairly high temperatures (−1.8 to −3.8 °C (28.8 to 25.2 °F)), resulting in injury. Since the 1970s, P. syringae has been implicated as an atmospheric “biological ice nucleator”, with airborne bacteria serving as cloud condensation nuclei. Recent evidence has suggested the species plays a larger role than previously thought in producing rain and snow. They have also been found in the cores of hailstones, aiding in bioprecipitation. These INA proteins are also used in making artificial snow.
- Maki, Leroy (September 1974). “Ice Nucleation Induced by Pseudomonas syringae”. Applied Microbiology. 28 (3): 456–59. doi:10.1128/AEM.28.3.456-459.1974. PMC 186742. PMID 4371331.
- Palmer, Jason (25 May 2011). “Bacteria-rich hailstones add to ‘bioprecipitation’ idea”. BBC News.
- Robbins, Jim (24 May 2010). “From Trees and Grass, Bacteria That Cause Snow and Rain”. The New York Times.
Pseudomonas syringae pathogenesis is dependent on effector proteins secreted into the plant cell by the bacterial type III secretion system. Nearly 60 different type III effector families encoded by hop genes have been identified in P. syringae. Type III effectors contribute to pathogenesis chiefly through their role in suppressing plant defense. Owing to early availability of the genome sequence for three P. syringae strains and the ability of selected strains to cause disease on well-characterized host plants, including Arabidopsis thaliana, Nicotiana benthamiana, and the tomato, P. syringae has come to represent an important model system for experimental characterization of the molecular dynamics of plant-pathogen interactions.
- “PPI home – Pseudomonas-Plant Interaction – Pseudomonas syringae Genome Resources Home Page”. Cornell University & National Science Foundation & USDA ARS. 3 September 2010. Retrieved 22 March 2022.
- Mansfield, John (2012). “Top 10 plant pathogenic bacteria in molecular plant pathology”. Molecular Plant Pathology. 13 (6): 614–29. doi:10.1111/j.1364-3703.2012.00804.x. PMC 6638704. PMID 22672649.
History
In 1961, Paul Hoppe of the U.S. Department of Agriculture studied a corn fungus by grinding up infected leaves each season, then applying the powder to test corn for the following season to track the disease. A surprise frost occurred that year, leaving peculiar results. Only plants infected with the diseased powder incurred frost damage, leaving healthy plants unfrozen. This phenomenon baffled scientists until graduate student Steven E. Lindow of the University of Wisconsin–Madison with D.C. Arny and C. Upper found a bacterium in the dried leaf powder in the early 1970s. Steven E. Lindow, now a plant pathologist at the University of California, Berkeley, found that when this particular bacterium was introduced to plants where it is originally absent, the plants became very vulnerable to frost damage. He went on to identify the bacterium as P. syringae, investigate the role of P. syringae in ice nucleation and in 1977, discover the mutant ice-minus strain. He was later successful at producing the ice-minus strain of P. syringae through recombinant DNA technology, as well.
- Parrott, Carolyn C. (1993). “Recombinant DNA to Protect Crops”. Archived from the original on 18 September 2012.
- Lindow, Steven E.; Arny, Deane C.; Upper, Christen D. (1 October 1982). “Bacterial Ice Nucleation: A Factor in Frost Injury to Plants”. Plant Physiology. American Society of Plant Biologists (ASPB). 70 (4): 1084–1089. doi:10.1104/pp.70.4.1084. ISSN 0032-0889. PMC 1065830. PMID 16662618.
- Hynes, H. Patricia (1989). “Biotechnology in agriculture: an analysis of selected technologies and policy in the United States” (PDF). Reproductive and Genetic Engineering. 2 (1): 39–49. Archived from the original (PDF) on 4 December 2014. Retrieved 3 September 2012.
Ice-minus bacteria is a common name given to a variant of the common bacterium Pseudomonas syringae (P. syringae). This strain of P. syringae lacks the ability to produce a certain surface protein, usually found on wild-type P. syringae. The “ice-plus” protein (INA protein, “Ice nucleation-active” protein) found on the outer bacterial cell wall acts as the nucleating centers for ice crystals.[Love, J.; Lesser, W. (April 1989). “The Potential Impact of Ice-Minus Bacteria as a Frost Protestant in New York Tree Fruit Production” (PDF). Northeastern Journal of Agricultural and Resource Economics. 18 (1): 26–34. doi:10.1017/S0899367X00000234.] This facilitates ice formation, hence the designation “ice-plus”. The ice-minus variant of P. syringae is a mutant, lacking the gene responsible for ice-nucleating surface protein production. This lack of surface protein provides a less favorable environment for ice formation. Both strains of P. syringae occur naturally, but recombinant DNA technology has allowed for the synthetic removal or alteration of specific genes, enabling the ice-minus strain to be created from the ice-plus strain in the lab.
Production
To systematically create the ice-minus strain of P. syringae, its ice-forming gene must be isolated, amplified, deactivated and reintroduced into P. syringae bacterium. The following steps are often used to isolate and generate ice-minus strains of P. syringae:
- Digest P. syringae’s DNA with restriction enzymes.
- Insert the individual DNA pieces into a plasmid. Pieces will insert randomly, allowing for different variations of recombinant DNA to be produced.
- Transform the bacterium Escherichia coli (E.coli) with the recombinant plasmid. The plasmid will be taken in by the bacteria, rendering it part of the organism’s DNA.
- Identify the ice-gene from the numerous newly developed E. coli recombinants. Recombinant E. coli with the ice-gene will possess the ice-nucleating phenotype, these will be “ice-plus”.
- With the ice nucleating recombinant identified, amplify the ice gene with techniques such as polymerase chain reactions (PCR).
- Create mutant clones of the ice gene through the introduction of mutagenic agents such as UV radiation to inactivate the ice gene, creating the “ice-minus” gene.
- Repeat previous steps (insert gene into plasmid, transform E. coli, identify recombinants) with the newly created mutant clones to identify the bacteria with the ice-minus gene. They will possess the desired ice-minus phenotype.
- Insert the ice-minus gene into normal, ice-plus P. syringae bacterium.
- Allow recombination to take place, rendering both ice-minus and ice-plus strains of P. syringae.
Economic importance
In the United States alone, it has been estimated that frost accounts for approximately $1 billion in crop damage each year.[citation needed] As P. syringae commonly inhabits plant surfaces, its ice nucleating nature incites frost development, freezing the buds of the plant and destroying the occurring crop. The introduction of an ice-minus strain of P. syringae to the surface of plants would incur competition between the strains. Should the ice-minus strain win out, the ice nucleate provided by P. syringae would no longer be present, lowering the level of frost development on plant surfaces at normal water freezing temperature – 0 °C (32 °F). Even if the ice-minus strain does not win out, the amount of ice nucleate present from ice-plus P. syringae would be reduced due to competition. Decreased levels of frost generation at normal water freezing temperature would translate into a lowered quantity of crops lost due to frost damage, rendering higher crop yields overall.
The ice nucleating nature of P. syringae incites frost development, freezing the buds of the plant and destroying the occurring crop. The introduction of an ice-minus strain of P. syringae to the surface of plants would reduce the amount of ice nucleate present, rendering higher crop yields. The recombinant form was developed as a commercial product known as Frostban. Field-testing of Frostban in 1987 was the first release of a genetically modified organism into the environment. The testing was very controversial and drove the formation of US biotechnology policy. Frostban was never marketed.
Controversy
At the time of Lindow’s work on ice-minus P. syringae, genetic engineering was considered to be very controversial. Jeremy Rifkin and his Foundation on Economic Trends (FET) sued the NIH in federal court to delay the field trials, arguing that NIH had failed to conduct an Environmental Impact Assessment and had failed to explore the possible effects “Ice-minus” bacteria might have on ecosystems and even global weather patterns.[Bratspies, Rebecca (2007). “Some Thoughts on the American Approach to Regulating Genetically Modified Organisms” (PDF). Kansas Journal of Law and Public Policy. 16 (3): 393. SSRN1017832.[dead link]][Maykuth, Andrew (January 10, 1986). “Genetic wonders to come: Some see boon, others calamity”. The Philadelphia Inquirer. Retrieved February 11, 2007.] Once approval was granted, both test fields were attacked by activist groups the night before the tests occurred: “The world’s first trial site attracted the world’s first field trasher”.[“GM crops: A bitter harvest?”. BBC News. June 14, 2002. Retrieved April 4, 2016.] The BBC quoted Andy Caffrey from Earth First!: “When I first heard that a company in Berkley was planning to release these bacteria Frostban in my community, I literally felt a knife go into me. Here once again, for a buck, science, technology and corporations were going to invade my body with new bacteria that hadn’t existed on the planet before. It had already been invaded by smog, by radiation, by toxic chemicals in my food, and I just wasn’t going to take it anymore.”[“GM crops: A bitter harvest?”. BBC News. June 14, 2002. Retrieved April 4, 2016.]
Rifkin’s successful legal challenge forced the Reagan Administration to more quickly develop an overarching regulatory policy to guide federal decision-making about agricultural biotechnology. In 1986, the Office of Science and Technology Policy issued the Coordinated Framework for Regulation of Biotechnology, which continues to govern US regulatory decisions.[Bratspies, Rebecca (2007). “Some Thoughts on the American Approach to Regulating Genetically Modified Organisms” (PDF). Kansas Journal of Law and Public Policy. 16 (3): 393. SSRN1017832.[dead link]] The controversy drove many biotech companies away from use of genetically engineering microorganisms in agriculture.[Baskin, Yvonne (1987). “Testing The Future”. Alicia Patterson Foundation. Retrieved February 11, 2007.]
- See also
- Bacterial ice-nucleation proteins
- Bacterial ice-nucleation proteins is a family of proteins that enable Gram-negative bacteria to promote nucleation of ice at relatively high temperatures (above -5C).
- Wolber PK, Green RL (October 1990). “Detection of bacteria by transduction of ice nucleation genes”. Trends in Biotechnology. 8 (10): 276–9. doi:10.1016/0167-7799(90)90195-4. PMID 1366726.
- Gurian-Sherman D, Lindow SE (November 1993). “Bacterial ice nucleation: significance and molecular basis”. FASEB Journal. 7 (14): 1338–43. doi:10.1096/fasebj.7.14.8224607. PMID 8224607. S2CID 12368098.
- These proteins are localised at the outer membrane surface and can cause frost damage to many plants. The primary structure of the proteins contains a highly repetitive domain that dominates the sequence. The domain comprises a number of 48-residue repeats, which themselves contain 3 blocks of 16 residues, the first 8 of which are identical. It is thought that the repetitive domain may be responsible for aligning water molecules in the seed crystal.
- See also Ice-minus bacteria
- This article incorporates text from the public domain Pfam and InterPro: IPR000258
- Category: Protein domains
- Bacterial ice-nucleation proteins is a family of proteins that enable Gram-negative bacteria to promote nucleation of ice at relatively high temperatures (above -5C).
- Bacterial ice-nucleation proteins
- External links
Genomics
Based on a comparative genomic and phylogenomic analysis of 494 complete genomes from the entire Pseudomonas genus, P. syringae does not form a monophyletic species in the strict sense, but a wider evolutionary group (34 genomes in total, organized into 3 subgroups) that includes other species as well. The core proteome of the P. syringae group comprised 2944 proteins, whereas the protein count and GC content of the strains of this group ranged between 4973 and 6026 (average: 5465) and between 58 and 59.3% (average: 58.6%), respectively.
- Nikolaidis, Marios; Mossialos, Dimitris; Oliver, Stephen G.; Amoutzias, Grigorios D. (August 2020). “Comparative Analysis of the Core Proteomes among the Pseudomonas Major Evolutionary Groups Reveals Species-Specific Adaptations for Pseudomonas aeruginosa and Pseudomonas chlororaphis”. Diversity. 12 (8): 289. doi:10.3390/d12080289.
Disease cycle
Pseudomonas syringae overwinters on infected plant tissues such as regions of necrosis or gummosis (sap oozing from wounds on the tree) but can also overwinter in healthy looking plant tissues. In the spring, water from rain or other sources will wash the bacteria onto leaves/blossoms where it will grow and survive throughout the summer. This is the epiphyte phase of P. syringae’s life cycle where it will multiply and spread but will not cause a disease. Once it enters the plant through a leaf’s stomata or necrotic spots on either leaves or woody tissue then the disease will start. The pathogen will then exploit and grow in intercellular space causing the leaf spots and cankers. P. syringae can also survive in temperatures slightly below freezing. These below freezing temperatures increase the severity of infection within trees like sour cherry, apricot, and peach.
- Kennelly, Megan M.; Cazorla, Francisco M.; de Vicente, Antonio; Ramos, Cayo; Sundin, George W. (January 2007). “Pseudomonas syringae Diseases of Fruit Trees: Progress Toward Understanding and Control”. Plant Disease. 91 (1): 4–17. doi:10.1094/PD-91-0004. ISSN 0191-2917. PMID 30781059.
- Jeong, R.-D.; Chu, E.-H.; Lee, G.W.; Park, J.M.; Park, H.-J. (24 March 2016). “Effect of gamma irradiation on Pseudomonas syringae pv. tomato DC3000 – short communication”. Plant Protection Science. 52 (2): 107–12. doi:10.17221/68/2015-pps. ISSN 1212-2580.
Epidemiology
Diseases caused by P. syringae tend to be favoured by wet, cool conditions—optimum temperatures for disease tend to be around 12–25 °C (54–77 °F), although this can vary according to the pathovar involved. The bacteria tend to be seed-borne, and are dispersed between plants by rain splash.
- Hirano, S S; Upper, C D (1990). “Population Biology and Epidemiology of Pseudomonas Syringae”. Annual Review of Phytopathology. 28: 155–77. doi:10.1146/annurev.py.28.090190.001103.
Although it is a plant pathogen, it can also live as a saprotroph in the phyllosphere when conditions are not favourable for disease. Some saprotrophic strains of P. syringae have been used as biocontrol agents against postharvest rots.
- Hirano, S. S.; Upper, C. D. (2000). “Bacteria in the Leaf Ecosystem with Emphasis on Pseudomonas syringae—a Pathogen, Ice Nucleus, and Epiphyte”. Microbiology and Molecular Biology Reviews. 64 (3): 624–53. doi:10.1128/MMBR.64.3.624-653.2000. PMC 99007. PMID 10974129.
- Janisiewicz WJ, Marchi A (1992). “Control of Storage Rots on Various Pear Cultivars with a Saprophytic Strain of Pseudomonas syringae”. Plant Disease. 76 (6): 555–60. doi:10.1094/pd-76-0555.
From Wikipedia, the free encyclopedia (Redirected from Saprotroph)
Saprotrophic nutrition or lysotrophic nutrition is a process of chemoheterotrophic extracellular digestion involved in the processing of decayed (dead or waste) organic matter. It occurs in saprotrophs, and is most often associated with fungi (for example Mucor) and soil bacteria. Saprotrophic microscopic fungi are sometimes called saprobes. Saprotrophic plants or bacterial flora are called saprophytes (sapro- ‘rotten material’ + -phyte ‘plant’), although it is now believed[citation needed] that all plants previously thought to be saprotrophic are in fact parasites of microscopic fungi or other plants. The process is most often facilitated through the active transport of such materials through endocytosis within the internal mycelium and its constituent hyphae.
- “saprotroph – definition of saprotroph in English from the Oxford dictionary”. OxfordDictionaries.com. Archived from the original on November 8, 2012. Retrieved 2016-01-20.
- “The Ecology of Story: Revealing Hidden Characters of the Forest”. April 25, 2020.
- Clegg & Mackean (2006, p. 296) states the purpose of saprotrophs and their internal nutrition, as well as the main two types of fungi that are most often referred to, as well as describes, visually, the process of saprotrophic nutrition through a diagram of hyphae, referring to the Rhizobium on damp, stale whole-meal bread or rotting fruit.
Various word roots relating to decayed matter (detritus, sapro-), eating and nutrition (-vore, -phage), and plants or life forms (-phyte, -obe) produce various terms, such as detritivore, detritophage, saprotroph, saprophyte, saprophage, and saprobe; their meanings overlap, although technical distinctions (based on physiologic mechanisms) narrow the senses. For example, usage distinctions can be made based on macroscopic swallowing of detritus (as an earthworm does) versus microscopic lysis of detritus (as a mushroom does).
Process
As matter decomposes within a medium in which a saprotroph is residing, the saprotroph breaks such matter down into its composites.
- Proteins are broken down into their amino acid composites through the breaking of peptide bonds by proteases.
- Lipids are broken down into fatty acids and glycerol by lipases.
- Starch is broken down into pieces of simple disaccharides by amylases.
- Cellulose, a major portion of plant cells, and therefore a major constituent of decaying matter is broken down into glucose
- Clegg & Mackean (2006, p. 296), fig 14.16—Diagram detailing the re-absorption of substrates within the hypha.
These products are re-absorbed into the hypha through the cell wall by endocytosis and passed on throughout the mycelium complex. This facilitates the passage of such materials throughout the organism and allows for growth and, if necessary, repair.
- Clegg & Mackean (2006, p. 296) states the purpose of saprotrophs and their internal nutrition, as well as the main two types of fungi that are most often referred to, as well as describes, visually, the process of saprotrophic nutrition through a diagram of hyphae, referring to the Rhizobium on damp, stale whole-meal bread or rotting fruit.
Conditions
In order for a saprotrophic organism to facilitate optimal growth and repair, favourable conditions and nutrients must be present.
- Clegg & Mackean (2006, p. 296), fig 14.17—A diagram explaining the optimal conditions needed for successful growth and repair.
Optimal conditions refers to several conditions which optimise the growth of saprotrophic organisms, such as;
- Presence of water: 80–90% of the mass of the fungi is water, and the fungi require excess water for absorption due to the evaporation of internally retained water.
- Presence of oxygen: Very few saprotrophic organisms can endure anaerobic conditions as evidenced by their growth above media such as water or soil.
- Neutral-acidic pH: The condition of neutral or mildly acidic conditions under pH 7 are required.
- Low-medium temperature: The majority of saprotrophic organisms require temperatures between 1 °C and 35 °C (33.8 °F and 95 °F), with optimum growth occurring at 25 °C (77 °F).
The majority of nutrients taken in by such organisms must be able to provide carbon, proteins, vitamins and, in some cases, ions. Due to the carbon composition of the majority of organisms, dead and organic matter provide rich sources of disaccharides and polysaccharides such as maltose and starch, and of the monosaccharide glucose.
- Clegg & Mackean (2006, p. 296) states the purpose of saprotrophs and their internal nutrition, as well as the main two types of fungi that are most often referred to, as well as describes, visually, the process of saprotrophic nutrition through a diagram of hyphae, referring to the Rhizobium on damp, stale whole-meal bread or rotting fruit.
In terms of nitrogen-rich sources, saprotrophs require combined protein for the creation of proteins, which is facilitated by the absorption of amino acids, and usually taken from rich soil. Although both ions and vitamins are rare, thiamine or ions such as potassium, phosphorus, and magnesium aid the growth of the mycelium.
- Clegg & Mackean (2006, p. 296) states the purpose of saprotrophs and their internal nutrition, as well as the main two types of fungi that are most often referred to, as well as describes, visually, the process of saprotrophic nutrition through a diagram of hyphae, referring to the Rhizobium on damp, stale whole-meal bread or rotting fruit.
- See also
- Further reading
- Clegg, C. J.; Mackean, D. G. (2006). Advanced Biology: Principles and Applications (2nd ed.). Hodder Publishing.
- Zmitrovich, I. V.; Wasser, S. P.; Ţura, D. (2014). “Wood-inhabiting fungi” (PDF). In Misra, J. K.; Tewari, J. P.; Deshmukh, S. K.; Vágvölgyi, C. (eds.). Fungi from Different Substrates. N. Y.: CRC Press, Taylor and Francis group. pp. 17–74.
Ecology: Modelling ecosystems: Trophic components |
---|
Ecology: Modelling ecosystems: Other components |
---|
Mechanisms of pathogenicity
The mechanisms of P. syringae pathogenicity can be separated into several categories: ability to invade a plant, ability to overcome host resistance, biofilm formation, and production of proteins with ice-nucleating properties.
- Ichinose, Yuki; Taguchi, Fumiko; Mukaihara, Takafumi (2013). “Pathogenicity and virulence factors of Pseudomonas syringae”. J Gen Plant Pathol. 79 (5): 285–296. doi:10.1007/s10327-013-0452-8. S2CID 17519705.
Ability to invade plants
Planktonic P. syringae is able to enter plants using its flagella and pili to swim towards a target host. It enters the plant via wounds of natural opening sites, as it is not able to breach the plant cell wall. An example of this is the partnership with the leaf-mining fly Scaptomyza flava, which creates holes in leaves during oviposition that the pathogen can take advantage of. The role of taxis in P. syringae has not been well-studied, but the bacteria are thought to use chemical signals released by the plant to find their host and cause infection.
- Ichinose, Yuki; Taguchi, Fumiko; Mukaihara, Takafumi (2013). “Pathogenicity and virulence factors of Pseudomonas syringae”. J Gen Plant Pathol. 79 (5): 285–296. doi:10.1007/s10327-013-0452-8. S2CID 17519705.
- Groen, Simon C.; Humphrey, Parris T.; Chevasco, Daniela; Ausubel, Frederick M.; Pierce, Naomi E.; Whiteman, Noah K. (1 January 2016). “Pseudomonas syringae enhances herbivory by suppressing the reactive oxygen burst in Arabidopsis”. Journal of Insect Physiology. plant-reprogramming insects: from effector molecules to ecosystem engineering. 84: 90–102. doi:10.1016/j.jinsphys.2015.07.011. ISSN 0022-1910. PMC 4721946. PMID 26205072.
Overcoming host resistance
Effectors
Pseudomonas syringae isolates carry a range of virulence factors called type III secretion system (T3SS) effector proteins. These proteins primarily function to cause disease symptoms and manipulate the host’s immune response to facilitate infection. The major family of T3SS effectors in P. syringae is the hrp gene cluster, coding for the Hrp secretion apparatus.
- Ichinose, Yuki; Taguchi, Fumiko; Mukaihara, Takafumi (2013). “Pathogenicity and virulence factors of Pseudomonas syringae”. J Gen Plant Pathol. 79 (5): 285–296. doi:10.1007/s10327-013-0452-8. S2CID 17519705.
Hop effectors
HopZ1s are type III effectors which interfere with the Glycine max 2-hydroxyisoflavanone dehydratase (GmHID1). HopZ1b degrades daidzein after production, reducing concentrations and thus reducing the immunity it provides the plant.
- Bauters, Lander; Stojilković, Boris; Gheysen, Godelieve (19 August 2021). “Pathogens pulling the strings: Effectors manipulating salicylic acid and phenylpropanoid biosynthesis in plants”. Molecular Plant Pathology. 22 (11): 1436–1448. doi:10.1111/mpp.13123. ISSN 1464-6722. PMC 8518561. PMID 34414650.
Elicitors
Pst DC3000 produces a PsINF1, the INF1 in P. syringae. Hosts respond with autophagy upon detection of this elicitor. Liu et al. 2005 finds this to be the only alternative to mass hypersensitivity leading to mass programmed cell death.
- Yang, Meng; Ismayil, Asigul; Liu, Yule (29 September 2020). “Autophagy in Plant-Virus Interactions”. Annual Review of Virology. Annual Reviews. 7 (1): 403–419. doi:10.1146/annurev-virology-010220-054709. ISSN 2327-056X. PMID 32530794. S2CID 219621432.
Phytotoxins
The pathogens also produce phytotoxins which injure the plant and can suppress the host immune system. One such phytotoxin is coronatine, found in pathovars Pto and Pgl.
- Ichinose, Yuki; Taguchi, Fumiko; Mukaihara, Takafumi (2013). “Pathogenicity and virulence factors of Pseudomonas syringae”. J Gen Plant Pathol. 79 (5): 285–296. doi:10.1007/s10327-013-0452-8. S2CID 17519705.
Coronatine (COR) is a toxin produced by the bacterium Pseudomonas syringae. It is involved in causing stomata to re-open after they close in response to pathogen-associated molecular patterns, as well as interfering with the responses mediated by salicylic acid after the infection has begun. It consists of coronafacic acid (CFA), which is an analog of methyl jasmonic acid (MeJA), and coronamic acid (CMA), joined by an amide bond between the acid group of CFA and the amino group of CMA.
- Melotto, Maeli; Underwood, William; He, Sheng Yang (2008). “Role of Stomata in Plant Innate Immunity and Foliar Bacterial Diseases”. Annual Review of Phytopathology. 46: 101–22. doi:10.1146/annurev.phyto.121107.104959. PMC 2613263. PMID 18422426.
Biofilm formation
Pseudomonas syringae produces polysaccharides which allow it to adhere to the surface of plant cells. It also releases quorum sensing molecules, which allows it to sense the presence of other bacterial cells nearby. If these molecules pass a threshold level, the bacteria change their pattern of gene expression to form a biofilm and begin expression of virulence-related genes. The bacteria secrete highly viscous compounds such as polysaccharides and DNA to create a protective environment in which to grow.
- Ichinose, Yuki; Taguchi, Fumiko; Mukaihara, Takafumi (2013). “Pathogenicity and virulence factors of Pseudomonas syringae”. J Gen Plant Pathol. 79 (5): 285–296. doi:10.1007/s10327-013-0452-8. S2CID 17519705.
Ice-nucleating properties
Pseudomonas syringae—more than any mineral or other organism—is responsible for the surface frost damage in plants exposed to the environment. For plants without antifreeze proteins, frost damage usually occurs between −4 and −12 °C (25 and 10 °F) as the water in plant tissue can remain in a supercooled liquid state. P. syringae can cause water to freeze at temperatures as high as −1.8 °C (28.8 °F), but strains causing ice nucleation at lower temperatures (down to −8 °C (18 °F)) are more common. The freezing causes injuries in the epithelia and makes the nutrients in the underlying plant tissues available to the bacteria.[citation needed]
- Hirano, Susan S.; Upper, Christen D. (1995). “Ecology of Ice Nucleation – Active Bacteria”. In Lee, Richard E.; Warren, Gareth J.; Gusta, L. V. (eds.). Biological Ice Nucleation and Its Applications. St. Paul, Minnesota: American Phytopathological Society. pp. 41–61. ISBN 978-0-89054-172-2.
- Maki, LR; Galyan, EL; Chang-Chien, MM; Caldwell, DR (1974). “Ice nucleation induced by Pseudomonas syringae“. Applied Microbiology. 28 (3): 456–59. doi:10.1128/AEM.28.3.456-459.1974. PMC 186742. PMID 4371331.
- Fall, Ray; Wolber, Paul K. (1995). “Biochemistry of Bacterial Ice Nuclei”. In Lee, Richard E.; Warren, Gareth J.; Gusta, L. V. (eds.). Biological Ice Nucleation and Its Applications. St. Paul, Minnesota: American Phytopathological Society. pp. 63–83. ISBN 978-0-89054-172-2.
Pseudomonas syringae has ina (ice nucleation-active) genes that make INA proteins which translocate to the outer bacterial membrane on the surface of the bacteria, where the proteins act as nuclei for ice formation. Artificial strains of P. syringae known as ice-minus bacteria have been created to reduce frost damage.[citation needed]
- Fall, Ray; Wolber, Paul K. (1995). “Biochemistry of Bacterial Ice Nuclei”. In Lee, Richard E.; Warren, Gareth J.; Gusta, L. V. (eds.). Biological Ice Nucleation and Its Applications. St. Paul, Minnesota: American Phytopathological Society. pp. 63–83. ISBN 978-0-89054-172-2.
Pseudomonas syringae has been found in the center of hailstones, suggesting the bacterium may play a role in Earth’s hydrological cycle.
- Palmer, Jason (25 May 2011). “Bacteria-rich hailstones add to ‘bioprecipitation’ idea”. BBC News.
Management
Currently there is not a 100% effective way to eradicate P. syringae from a field. The most common way to control this pathogen is to spray bactericides with copper compounds or other heavy metals that can be combined with fungicides or other pest control chemicals. Chemical treatments with fixed copper such as Bordeaux, copper hydroxide, and cupric sulfate are used to stop the spread of P. syringae by killing the bacteria while it is in the epiphyte stage on leaves, or woody parts of trees – however resistant P. syringae strains do exist. Spraying antibiotics such as streptomycin and organic bactericides is another way to control P. syringae but is less common than the methods listed above.
- Cazorla, Francisco M.; Arrebola, Eva; Sesma, Ane; Pérez-García, Alejandro; Codina, Juan C.; Murillo, Jesús; de Vicente, Antonio (2002). “Copper Resistance in Pseudomonas syringae Strains Isolated from Mango Is Encoded Mainly by Plasmids”. Phytopathology. Scientific Societies. 92 (8): 909–916. doi:10.1094/phyto.2002.92.8.909. ISSN 0031-949X. PMID 18942971.
- Bashan, Yoav (1997), “Alternative Strategies for Controlling Plant Diseases Caused by Pseudomonas syringae”, in Rudolph, K.; Burr, T. J.; Mansfield, J. W.; Stead, D. (eds.), Pseudomonas Syringae Pathovars and Related Pathogens, Developments in Plant Pathology, vol. 9, Springer Netherlands, pp. 575–83, doi:10.1007/978-94-011-5472-7_105, ISBN 978-94-010-6301-2
New research has shown that adding ammonium (NH4+) nutrition to tomato plants can cause a metabolic change leading to resistance against Pseudomonas syringae. This “ammonium syndrome” causes nutrient imbalances in the plant and therefore triggers a defense response against the pathogen.
- González-Hernández, Ana Isabel; Fernández-Crespo, Emma; Scalschi, Loredana; Hajirezaei, Mohammad-Reza; von Wirén, Nicolaus; García-Agustín, Pilar; Camañes, Gemma (August 2019). “Ammonium mediated changes in carbon and nitrogen metabolisms induce resistance against Pseudomonas syringae in tomato plants”. Journal of Plant Physiology. 239: 28–37. doi:10.1016/j.jplph.2019.05.009. PMID 31177028. S2CID 182543294.
Strict hygiene practices used in orchards along with pruning in early spring and summer were proven to make the trees more resistant to P. syringae. Cauterizing cankers found on orchard trees can save the tree’s life by stopping the infection from spreading.
- “Diseases Caused by Pseudomonas syringe”. Pacific Northwest Pest Management Handbooks. 10 September 2015. Retrieved 11 December 2019.
Breeding plants for resistance is another somewhat effective way to avoid P. syringae. It has been successful in the cherry rootstock with Pseudomonas syringae pv. syringae, but so far, no other species are 100% resistant to this pathogen. Resistance breeding is a slow process, especially in trees. Unfortunately, P. syringae bacteria can adapt genetically to infect resistant plants, and the process for resistance breeding has to start over again.
A combination treatment of bacteriophage and carvacrol shows promise in control of both the planktonic and biofilm forms.
- Ni, Peien; Wang, Lei; Deng, Bohan; Jiu, Songtao; Ma, Chao; Zhang, Caixi; Almeida, Adelaide; Wang, Dapeng; Xu, Wenping; Wang, Shiping (2 June 2020). “Combined Application of Bacteriophages and Carvacrol in the Control of Pseudomonas syringae pv. actinidiae Planktonic and Biofilm Forms”. Microorganisms. MDPI AG. 8 (6): 837. doi:10.3390/microorganisms8060837. ISSN 2076-2607. PMC 7356356. PMID 32498472.
Pathovars
Following ribotype analysis, incorporation of several pathovars of P. syringae into other species was proposed (see P. amygdali, ‘P. tomato’, P. coronafaciens, P. avellanae, ‘P. helianthi’, P. tremae, P. cannabina, and P. viridiflava).
- Gardan, L.; Shafik, H.; Belouin, S.; Broch, R.; Grimont, F.; Grimont, P. A. D. (1999). “DNA relatedness among the pathovars of Pseudomonas syringae and description of Pseudomonas tremae sp. nov. and Pseudomonas cannabina sp. nov. (ex Sutic and Dowson 1959)”. International Journal of Systematic Bacteriology. 49 (2): 469–78. doi:10.1099/00207713-49-2-469. PMID 10319466.
According to this schema, the remaining pathovars are:
- P. s. pv. aceris attacks maple Acer species.
- P. s. pv. actinidiae attacks kiwifruit Actinidia deliciosa.
- P. s. pv. aesculi attacks horse chestnut Aesculus hippocastanum, causing bleeding canker.
- P. s. pv. aptata attacks beets Beta vulgaris.
- P. s. pv. atrofaciens attacks wheat Triticum aestivum.
- P. s. pv. dysoxylis attacks the kohekohe tree Dysoxylum spectabile.
- P. s. pv. glycinea attacks soybean Glycine max, causing bacterial blight of soybean.
- P. s. pv. japonica attacks barley Hordeum vulgare.
- P. s. pv. lapsa attacks wheat Triticum aestivum.
- P. s. pv. panici attacks Panicum grass species.
- P. s. pv. papulans attacks crabapple Malus sylvestris species.
- P. s. pv. persicae attacks nectarine and peach.
- P. s. pv. phaseolicola causes halo blight of beans.
- P. s. pv. pisi attacks peas Pisum sativum.
- P. s. pv. syringae attacks Syringa, Prunus, and Phaseolus species.
- P. s. pv. tomato attacks tomato.
- “Pseudomonas syringae pv. actinidiae (PSDMAK)[Overview]”. Global Database. EPPO (European and Mediterranean Plant Protection Organization). 7 February 2008. Retrieved 13 July 2021.
- Di Lallo G, Evangelisti M, Mancuso F, Ferrante P, Marcelletti S, Tinari A, Superti F, Migliore L, D’Addabbo P, Frezza D, Scortichini M, Thaller MC (2014). “Isolation and partial characterization of bacteriophages infecting Pseudomonas syringae pv. actinidiae, causal agent of kiwifruit bacterial canker” (PDF). J. Basic Microbiol. 54 (11): 1210–21. doi:10.1002/jobm.201300951. hdl:2108/92348. PMID 24810619. S2CID 11456671.
- “Bleeding Canker of Horse Chestnut”. UK Forestry Commission. Archived from the original on 17 December 2010. Retrieved 24 January 2011.
- Bennett, J. Michael; Hicks, Dale R.; Naeve, Seth L.; Bennett, Nancy Bush (2014). The Minnesota Soybean Field Book (PDF). St Paul, MN: University of Minnesota Extension. p. 84. Archived from the original (PDF) on 30 September 2013. Retrieved 21 February 2016.
- “Pseudomonas syringae pv. persicae (PSDMPE)[Overview]”. Global Database. EPPO (European and Mediterranean Plant Protection Organization). 28 January 2001. Retrieved 22 March 2022.
However, many of the strains for which new species groupings were proposed continue to be referred to in the scientific literature as pathovars of P. syringae, including pathovars tomato, phaseolicola, and maculicola. Pseudomonas savastanoi was once considered a pathovar or subspecies of P. syringae, and in many places continues to be referred to as P. s. pv. savastanoi, although as a result of DNA-relatedness studies, it has been instated as a new species.[33] It has three host-specific pathovars: P. s. fraxini (which causes ash canker), P. s. nerii (which attacks oleander), and P. s. oleae (which causes olive knot).
- Gardan, L.; Shafik, H.; Belouin, S.; Broch, R.; Grimont, F.; Grimont, P. A. D. (1999). “DNA relatedness among the pathovars of Pseudomonas syringae and description of Pseudomonas tremae sp. nov. and Pseudomonas cannabina sp. nov. (ex Sutic and Dowson 1959)”. International Journal of Systematic Bacteriology. 49 (2): 469–78. doi:10.1099/00207713-49-2-469. PMID 10319466.
Determinants of host specificity
A combination of the pathogen’s effector genes and the plant’s resistance genes is thought to determine which species a particular pathovar can infect. Plants can develop resistance to a pathovar by recognising pathogen-associated molecular patterns (PAMPs) and launching an immune response. These PAMPs are necessary for the microbe to function, so cannot be lost, but the pathogen may find ways to suppress this immune response, leading to an evolutionary arms race between the pathogen and the host.
- Baltrus, David; Nishimura, Marc; Dougherty, Kevin; Biswas, Surojit; Mukhtar, M. Shahid; Vicente, Joana; Holub, Eric; Jeffery, Dangl (2012). “The Molecular Basis of Host Specialization in Bean Pathovars of Pseudomonas syringae” (PDF). Molecular Plant-Microbe Interactions. 25 (7): 877–88. doi:10.1094/mpmi-08-11-0218. PMID 22414441.
- Ichinose, Yuki; Taguchi, Fumiko; Mukaihara, Takafumi (2013). “Pathogenicity and virulence factors of Pseudomonas syringae”. J Gen Plant Pathol. 79 (5): 285–296. doi:10.1007/s10327-013-0452-8. S2CID 17519705.
Pseudomonas syringae as a model system
Owing to early availability of genome sequences for P. syringae pv. tomato strain DC3000, P. syringae pv. syringae strain B728a, and P. syringae pv. phaseolicola strain 1448A, together with the ability of selected strains to cause disease on well-characterized host plants such as Arabidopsis thaliana, Nicotiana benthamiana, and tomato, P. syringae has come to represent an important model system for experimental characterization of the molecular dynamics of plant-pathogen interactions. The P. syringae experimental system has been a source of pioneering evidence for the important role of pathogen gene products in suppressing plant defense. The nomenclature system developed for P. syringae effectors has been adopted by researchers characterizing effector repertoires in other bacteria, and methods used for bioinformatic effector identification have been adapted for other organisms. In addition, researchers working with P. syringae have played an integral role in the Plant-Associated Microbe Gene Ontology working group, aimed at developing gene ontology terms that capture biological processes occurring during the interactions between organisms, and using the terms for annotation of gene products.
- Mansfield, John W. (2009). “From bacterial avirulence genes to effector functions via thehrpdelivery system: an overview of 25 years of progress in our understanding of plant innate immunity”. Molecular Plant Pathology. 10 (6): 721–34. doi:10.1111/j.1364-3703.2009.00576.x. PMC 6640528. PMID 19849780.
- Tobe, T.; Beatson, S. A.; Taniguchi, H.; Abe, H.; Bailey, C. M.; Fivian, A.; Younis, R.; Matthews, S.; et al. (2006). “An extensive repertoire of type III secretion effectors in Escherichia coli O157 and the role of lambdoid phages in their dissemination”. Proceedings of the National Academy of Sciences. 103 (40): 14941–6. Bibcode:2006PNAS..10314941T. doi:10.1073/pnas.0604891103. PMC 1595455. PMID 16990433.
- Torto-Alalibo, T.; Collmer, C. W.; Gwinn-Giglio, M.; Lindeberg, M.; Meng, S.; Chibucos, M. C.; Tseng, T.-T.; Lomax, J.; et al. (2010). “Unifying Themes in Microbial Associations with Animal and Plant Hosts Described Using the Gene Ontology”. Microbiology and Molecular Biology Reviews. 74 (4): 479–503. doi:10.1128/MMBR.00017-10. PMC 3008171. PMID 21119014
Pseudomonas syringae pv. tomato strain DC3000 and Arabidopsis thaliana
As mentioned above, the genome of P. syringae pv. tomato DC3000 has been sequenced, and approximately 40 Hop (Hrp Outer Protein) effectors – pathogenic proteins that attenuate the host cell – have been identified. These 40 effectors are not recognized by A. thaliana thus making P. syringae pv. tomato DC3000 virulent against it – that is, P. syringae pv. tomato DC3000 is able to infect A. thaliana – thus A. thaliana is susceptible to this pathogen.[citation needed]
- Buell CR, Joardar V, Lindeberg M, Selengut J, Paulsen IT, Gwinn ML, et al. (2003). “The complete genome sequence of the Arabidopsis and tomato pathogen Pseudomonas syringae pv. tomato DC3000”. Proc. Natl. Acad. Sci. U.S.A. 100 (18): 10181–86. Bibcode:2003PNAS..10010181B. doi:10.1073/pnas.1731982100. PMC 193536. PMID 12928499.
- Petnicki-Ocwieja T, Schneider DJ, Tam VC, Chancey ST, Shan L, Jamir Y, Schechter LM, Janes MD, Buell CR, Tang X, Collmer A, Alfano JR (2002). “Genomewide identification of proteins secreted by the Hrp type III protein secretion system of Pseudomonas syringae pv. tomato DC3000”. Proc. Natl. Acad. Sci. U.S.A. 99 (11): 7652–57. Bibcode:2002PNAS…99.7652P. doi:10.1073/pnas.112183899. PMC 124312. PMID 12032338.
Many gene-for-gene relationships have been identified using the two model organisms, P. syringae pv. tomato strain DC3000 and Arabidopsis. The gene-for-gene relationship describes the recognition of pathogenic avirulence (avr) genes by host resistance genes (R-genes). P. syringae pv. tomato DC3000 is a useful tool for studying avr: R-gene interactions in A. thaliana because it can be transformed with avr genes from other bacterial pathogens, and furthermore, because none of the endogenous hops genes is recognized by A. thaliana, any observed avr recognition identified using this model can be attributed to recognition of the introduced avr by A. thaliana. The transformation of P. syringae pv. tomato DC3000 with effectors from other pathogens have led to the identification of many R-genes in Arabidopsis to further advance knowledge of plant pathogen interactions.
- Hinsch, M; Staskawicz B. (9 January 1996). “Identification of a new Arabidopsis disease resistance locus, RPs4, and cloning of the corresponding avirulence gene, avrRps4, from Pseudomonas syringae pv. pisi”. Molecular Plant-Microbe Interactions. 9 (1): 55–61. doi:10.1094/mpmi-9-0055. PMID 8589423.
Examples of avr genes in P. syringae DC3000 and A. thaliana R-genes that recognize them
Avr gene | A. thaliana R-gene |
---|---|
AvrB | RPM1 |
AvrRpm1 | RPM1 |
AvrRpt2 | RPS2 |
AvrRps4 | RPS4 |
AvrRps6 | RPS6 |
AvrPphB * | RPS5/RESISTANCE TO PSEUDOMONAS SYRINGAE 5 (see also Arabidopsis thaliana § RPS5) * |
Pottinger, Sarah E.; Innes, Roger W. (25 August 2020). “RPS5-Mediated Disease Resistance: Fundamental Insights and Translational Applications”. Annual Review of Phytopathology. Annual Reviews. 58 (1): 139–160. doi:10.1146/annurev-phyto-010820-012733. ISSN 0066-4286. PMID 32284014. S2CID 215757180.
Wang, Yan; Tyler, Brett M.; Wang, Yuanchao (8 September 2019). “Defense and Counterdefense During Plant-Pathogenic Oomycete Infection”. Annual Review of Microbiology. Annual Reviews. 73 (1): 667–696. doi:10.1146/annurev-micro-020518-120022. ISSN 0066-4227. PMID 31226025. S2CID 195259901.
The Dynamin-related protein 2b/drp2b gene in A. thaliana is not directly an immunity gene, but by helping move external material into the intracellular network is indirectly related, and some mutants increase susceptibility.
- Ben Khaled, Sara; Postma, Jelle; Robatzek, Silke (4 August 2015). “A Moving View: Subcellular Trafficking Processes in Pattern Recognition Receptor–Triggered Plant Immunity”. Annual Review of Phytopathology. Annual Reviews. 53 (1): 379–402. doi:10.1146/annurev-phyto-080614-120347. ISSN 0066-4286. PMID 26243727.
Pseudomonas syringae pv. tomato strain DC3000, its derivatives, and its tomato host
As its name suggests, P. syringae pv. tomato DC3000 (Pst DC3000) is virulent to tomato (Solanum lycopersicum). However, the tomato cultivar Rio Grande-PtoR (RG-PtoR), harboring the resistance gene Pto, recognizes key effectors secreted by Pst DC3000, making it resistant to the bacteria. Studying the interactions between the Pto-expressing tomato lines and Pst DC3000 and its pathovars is a powerful system for understanding plant-microbe interactions.
- Martin GB, Williams JG, Tanksley SD (1991). “Rapid identification of markers linked to a Pseudomonas resistance gene in tomato by using random primers and near-isogenic lines”. Proc. Natl. Acad. Sci. 88 (6): 2336–40. Bibcode:1991PNAS…88.2336M. doi:10.1073/pnas.88.6.2336. PMC 51226. PMID 2006172.
- Schwizer S, Kraus CM, Dunham DM, Zheng Y, Fernandez-Pozo N, Pombo MA, et al. (2017). “The tomato kinase Pti1 contributes to production of reactive oxygen species in response to two flagellin-derived peptides and promotes resistance to Pseudomonas syringae infection” (PDF). Molecular Plant-Microbe Interactions. 30 (9): 725–38. doi:10.1094/MPMI-03-17-0056-R. PMID 28535079.
- Xiu-Fang X, He SY (2013). “Pseudomonas syringae pv. Tomato DC3000: A Model Pathogen for Probing Disease Susceptibility and Hormone Signaling in Plants”. Annu. Rev. Phytopathol. 51: 473–98. doi:10.1146/annurev-phyto-082712-102321. PMID 23725467.
Like other plants, the tomato has a two-tier pathogen defense system. The first and more universal line of plant defense, pattern-triggered immunity (PTI), is activated when plant pattern recognition receptors (PRRs) on the cell surface bind to pathogen-associated molecular patterns (PAMPs). The other branch of plant immunity, effector-triggered immunity (ETI), is triggered when intracellular (Nucleotide-binding site, Leucine-rich repeat) NB-LRR proteins bind to an effector, a molecule specific to a particular pathogen. ETI is generally more severe than PTI, and when a threshold of defense activation is reached, it can trigger a hypersensitive response (HR), which is purposeful death of host tissue to prevent the spread of infection. Two key effectors secreted by Pst DC3000 are AvrPto and AvrPtoB, which initiate ETI by binding the Pto/Prf receptor complex in Pto-expressing tomato lines like RG-PtoR.
- Jones JD, Dangl J (2006). “The plant immune system”. Nature. 444 (7117): 323–29. Bibcode:2006Natur.444..391Y. doi:10.1038/nature05281. PMID 17051149. S2CID 4419198.
- Kim YJ, Lin NC, Martin GB (2002). “Two distinct Pseudomonas effector proteins interact with the Pto kinase and activate plant immunity”. Cell. 109 (5): 589–598. doi:10.1016/S0092-8674(02)00743-2. PMID 12062102. S2CID 16848405.
Pst DC3000 has been modified to create the mutant strain Pst DC3000∆avrPto∆avrPtoB (Pst DC3000∆∆), which expresses neither AvrPto nor AvrPtoB. By infecting RG-PtoR with Pst DC3000∆∆, ETI to the pathogen is not triggered due to the absence of the main effectors recognized by the Pto/Prf complex. In the lab this is highly valuable, as using Pst DC3000∆∆ allows researchers to study the function of PTI-candidate genes in RG-PtoR, which would otherwise be masked by ETI.
- Kim YJ, Lin NC, Martin GB (2002). “Two distinct Pseudomonas effector proteins interact with the Pto kinase and activate plant immunity”. Cell. 109 (5): 589–598. doi:10.1016/S0092-8674(02)00743-2. PMID 12062102. S2CID 16848405.
- Lin N, Martin GB (2005). “An avrPto/avrPtoB Mutant of Pseudomonas syringae pv. tomato DC3000 Does Not Elicit Pto-Mediated Resistance and Is Less Virulent on Tomato”. Molecular Plant-Microbe Interactions. 18 (1): 43–51. doi:10.1094/MPMI-18-0043. PMID 15672817.
- Martin GB (2011). “Suppression and Activation of the Plant Immune System by Pseudomonas syringae Effectors AvrPto and AvrPtoB”. Effectors in Plant–Microbe Interactions. pp. 123–54. ISBN 9781119949138.
- Roberts R, Mainiero S, Powell AF, Liu AE, Shi K, Hind SR, et al. (2019). “Natural variation for unusual host responses and flagellin mediated immunity against Pseudomonas syringae in genetically diverse tomato accessions”. New Phytologist. 223 (1): 447–61. doi:10.1111/nph.15788. PMID 30861136.
Another useful DC3000 derivative is Pst DC3000∆avrPto∆avrPtoB∆fliC (Pst DC3000∆∆∆). Like Pst DC3000∆∆, this strain does not express AvrPto and AvrPtoB, but it also has an additional knock-out for fliC, the gene encoding flagellin, whose fragments serve as main PAMPs required for tomato PTI. By comparing plants within the same line that have been infected with either Pst DC3000∆∆ or Pst DC3000∆∆∆, researchers can determine if genes of interest are important to the flagellin recognition pathway of PTI.
- Rosli HG, Zheng Y, Pombo MA, Zhong S, Bombarely A, Fei Z, et al. (2013). “Transcriptomics-based screen for genes induced by flagellin and repressed by pathogen effectors identifies a cell wall-associated kinase involved in plant immunity”. Genome Biology. 14 (12): R139. doi:10.1186/gb-2013-14-12-r139. PMC 4053735. PMID 24359686.
- Kvitko BH, Park DH, Velasquez AC, Wei CF, Russell A, Martin GB, et al. (2009). “Deletions in the repertoire of Pseudomonas syringae pv. tomato DC3000 type III secretion effector genes reveal functional overlap among effectors”. PLOS Pathogens. 5 (4): e1000388. doi:10.1371/journal.ppat.1000388. PMC 2663052. PMID 19381254.
By treating CRISPR-induced tomato knockout mutants (in a RG-PtoR background) with Pst DC3000, Pst DC3000∆avrPto∆avrPtoB, or Pst DC3000∆avrPto∆avrPtoB∆fliC has led to the characterization of key components of the tomato immune system and continues to be used to further the field of tomato pathology.
Importance
Pseudomonas syringae has impacted many crop and orchard industries with its various pathovars.
P. s. pv. actinidiae
Mesarich et al. 2017 provides several libraries for transposon insertion sequencing of mutants of P. s. a.
- van Opijnen, Tim; Levin, Henry L. (23 November 2020). “Transposon Insertion Sequencing, a Global Measure of Gene Function”. Annual Review of Genetics. Annual Reviews. 54 (1): 337–365. doi:10.1146/annurev-genet-112618-043838. ISSN 0066-4197. PMC 9488914. PMID 32886545. S2CID 221504442.
The kiwifruit industry in New Zealand has suffered catastrophic losses since their first known outbreak in 2007 from P. syringae pv. actinidiae. New Zealand is second to Italy in the total volume of kiwifruit exports making an annual revenue of $NZ 1 billion, making it the most economically valuable export in the country. In 2014 the loss of exports alone was as high as NZ$930 million. Growers had to pay for treatments, and removal of infected vines along with suffering the loss of capital value in their orchards. For some, the orchard values went from NZ$450,000/ha to $70,000/ha after the outbreak, which is the price of bare land. The total loss of equity for the country of New Zealand was as high as NZ$2 billion.
- “Pseudomonas syringae pv. actinidiae (PSDMAK)[Overview]”. Global Database. EPPO (European and Mediterranean Plant Protection Organization). 7 February 2008. Retrieved 13 July 2021.
- Cameron, A.; Sarojini, V. (February 2014). “Pseudomonas syringae pv. actinidiae : chemical control, resistance mechanisms and possible alternatives”. Plant Pathology. 63 (1): 1–11. doi:10.1111/ppa.12066.
- Vanneste, Joel L. (4 August 2017). “The Scientific, Economic, and Social Impacts of the New Zealand Outbreak of Bacterial Canker of Kiwifruit (Pseudomonas syringae pv. actinidiae)”. Annual Review of Phytopathology. 55 (1): 377–99. doi:10.1146/annurev-phyto-080516-035530. ISSN 0066-4286. PMID 28613977.
Between 2010 and 2012 over 2,000 hectares (4,900 acres) of Italian kiwi orchards either were killed by P. syringae pv. actinidiae or were killed to contain the disease. The financial consequences for growers and their suppliers were severe, as were the economic consequences more widely.
- Donati, Irene; Cellini, Antonio; Sangiorgio, Daniela; Vanneste, Joel L.; Scortichini, Marco; Balestra, Giorgio M.; Spinelli, Francesco (3 January 2020). “Pseudomonas syringae pv. actinidiae: Ecology, Infection Dynamics and Disease Epidemiology”. Microbial Ecology. Springer Science and Business Media LLC. 80 (1): 81–102. doi:10.1007/s00248-019-01459-8. ISSN 0095-3628. PMC 7223186. PMID 31897570. S2CID 209542819.
See also
- Bioprecipitation
- Ice-minus bacteria
- Pseudomonas phage Φ6
- National Collection of Plant Pathogenic Bacteria
External links
Wikimedia Commons has media related to Pseudomonas syringae.
Wikispecies has information related to Pseudomonas syringae.
- Lavín, José L; Kiil, Kristoffer; Resano, Ohiana; Ussery, David W; Oguiza, José A (2007). “Comparative genomic analysis of two-component regulatory proteins in Pseudomonas syringae“. BMC Genomics. 8: 397. doi:10.1186/1471-2164-8-397. PMC 2222644. PMID 17971244.
- Type strain of Pseudomonas syringae at BacDive – the Bacterial Diversity Metadatabase
Crown gall
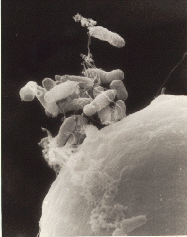
Agrobacterium tumefaciens
Agrobacterium radiobacter (more commonly known as Agrobacterium tumefaciens) is the causal agent of crown gall disease (the formation of tumours) in over 140 species of eudicots. It is a rod-shaped, Gram-negative soil bacterium. Symptoms are caused by the insertion of a small segment of DNA (known as the T-DNA, for ‘transfer DNA’, not to be confused with tRNA that transfers amino acids during protein synthesis), from a plasmid into the plant cell, which is incorporated at a semi-random location into the plant genome. Plant genomes can be engineered by use of Agrobacterium for the delivery of sequences hosted in T-DNA binary vectors.
- Smith EF, Townsend CO (April 1907). “A Plant-Tumor of Bacterial Origin”. Science. 25 (643): 671–3. Bibcode:1907Sci….25..671S. doi:10.1126/science.25.643.671. PMID 17746161.
- Buchanan RE (1965). “Proposal for rejection of the generic name Polymonas Lieske 1928”. International Bulletin of Bacteriological Nomenclature and Taxonomy. 15 (1): 43–44. doi:10.1099/00207713-15-1-43.
- Young JM, Kuykendall LD, Martínez-Romero E, Kerr A, Sawada H (January 2001). “A revision of Rhizobium Frank 1889, with an emended description of the genus, and the inclusion of all species of Agrobacterium Conn 1942 and Allorhizobium undicola de Lajudie et al. 1998 as new combinations: Rhizobium radiobacter, R. rhizogenes, R. rubi, R. undicola and R. vitis”. International Journal of Systematic and Evolutionary Microbiology. 51 (Pt 1): 89–103. doi:10.1099/00207713-51-1-89. PMID 11211278.
- Sawada H, Ieki H, Oyaizu H, Matsumoto S (1993). “Proposal for rejection of Agrobacterium tumefaciens and revised descriptions for the genus Agrobacterium and for Agrobacterium radiobacter and Agrobacterium rhizogenes”. Int J Syst Bacteriol. 43 (4): 694–702. doi:10.1099/00207713-43-4-694. PMID 8240952.
- Tindall BJ, et al. (Judicial Commission) (2014). “Judicial Opinion No. 94: Agrobacterium radiobacter (Beijerinck and van Delden 1902) Conn 1942 has priority over Agrobacterium tumefaciens (Smith and Townsend 1907) Conn 1942 when the two are treated as members of the same species based on the principle of priority and Rule 23a, Note 1 as applied to the corresponding specific epithets”. Int J Syst Evol Microbiol. 64 (Pt 10): 3590–3592. doi:10.1099/ijs.0.069203-0. PMID 25288664.
- “Rhizobium radiobacter (Agrobacterium tumefaciens) (Agrobacterium radiobacter)”. UniProt Taxonomy. Archived from the original on 2011-07-28. Retrieved 2010-06-30.
- “Taxonomy browser (Agrobacterium radiobacter K84)”. National Center for Biotechnology Information. Retrieved 7 December 2015.
- Chilton MD, Drummond MH, Merio DJ, Sciaky D, Montoya AL, Gordon MP, Nester EW (June 1977). “Stable incorporation of plasmid DNA into higher plant cells: the molecular basis of crown gall tumorigenesis”. Cell. 11 (2): 263–71. doi:10.1016/0092-8674(77)90043-5. PMID 890735. S2CID 7533482.
Agrobacterium tumefaciens | |
---|---|
Scientific classification | |
Domain: | Bacteria |
Phylum: | Pseudomonadota |
Class: | Alphaproteobacteria |
Order: | Hyphomicrobiales |
Family: | Rhizobiaceae |
Genus: | Agrobacterium |
Species: | A. radiobacter |
Binomial name | |
Agrobacterium radiobacter (Beijerinck and van Delden 1902) Conn 1942 (Approved Lists 1980) | |
Type strain | |
ATCC 23308[1] B6 CFBP 2413 HAMBI 1811 ICMP 5856 LMG 187 NCPPB 2437 | |
Synonyms | |
Bacillus radiobacter Beijerinck and van Delden 1902 Bacterium tumefaciens Smith and Townsend 1907 Beijerinckia fluminensis Döbereiner and Ruschel 1958 (Approved Lists 1980) Pseudomonas tumefaciens (Smith and Townsend 1907) Duggar 1909 Phytomonas tumefaciens (Smith and Townsend 1907) Bergey et al. 1923 Polymonas tumefaciens (Smith and Townsend 1900) Lieske 1928 Agrobacterium tumefaciens (Smith and Townsend 1907) Conn 1942 Rhizobium radiobacter (Beijerinck and van Delden 1902) Young et al. 2001 |
Agrobacterium tumefaciens is an Alphaproteobacterium of the family Rhizobiaceae, which includes the nitrogen-fixing legume symbionts. Unlike the nitrogen-fixing symbionts, tumor-producing Agrobacterium species are pathogenic and do not benefit the plant. The wide variety of plants affected by Agrobacterium makes it of great concern to the agriculture industry. Economically, A. tumefaciens is a serious pathogen of walnuts, grape vines, stone fruits, nut trees, sugar beets, horse radish, and rhubarb, and the persistent nature of the tumors or galls caused by the disease make it particularly harmful for perennial crops.
- Moore LW, Chilton WS, Canfield ML (January 1997). “Diversity of opines and opine-catabolizing bacteria isolated from naturally occurring crown gall tumors”. Applied and Environmental Microbiology. 63 (1): 201–7. Bibcode:1997ApEnM..63..201M. doi:10.1128/AEM.63.1.201-207.1997. PMC 1389099. PMID 16535484.
- “Crown Galls”. www.missouribotanicalgarden.org. Retrieved 2019-12-02.
Agrobacterium tumefaciens grows optimally at 28 °C (82 °F). The doubling time can range from 2.5–4h depending on the media, culture format, and level of aeration. At temperatures above 30 °C (86 °F), A. tumefaciens begins to experience heat shock which is likely to result in errors in cell division.
- Morton ER, Fuqua C (February 2012). “Laboratory maintenance of Agrobacterium”. Current Protocols in Microbiology. Chapter 1: Unit3D.1. doi:10.1002/9780471729259.mc03d01s24. ISBN 978-0471729259. PMC 3350319. PMID 22307549.
Conjugation
To be virulent, the bacterium contains a tumour-inducing plasmid (Ti plasmid or pTi) 200 kbp long, which contains the T-DNA and all the genes necessary to transfer it to the plant cell. Many strains of A. tumefaciens do not contain a pTi.
- Gordon JE, Christie PJ (December 2014). “The Agrobacterium Ti Plasmids”. Microbiology Spectrum. 2 (6). doi:10.1128/microbiolspec.PLAS-0010-2013. PMC 4292801. PMID 25593788.
Since the Ti plasmid is essential to cause disease, prepenetration events in the rhizosphere occur to promote bacterial conjugation – exchange of plasmids amongst bacteria. In the presence of opines, A. tumefaciens produces a diffusible conjugation signal called 30C8HSL or the Agrobacterium autoinducer[citation needed]. This activates the transcription factor TraR, positively regulating the transcription of genes required for conjugation[citation needed].
Infection methods
Agrobacterium tumefaciens infects the plant through its Ti plasmid. The Ti plasmid integrates a segment of its DNA, known as T-DNA, into the chromosomal DNA of its host plant cells. A. tumefaciens has flagella that allow it to swim through the soil towards photoassimilates that accumulate in the rhizosphere around roots. Some strains may chemotactically move towards chemical exudates from plants, such as acetosyringone and sugars, which indicate the presence of a wound in the plant through which the bacteria may enter. Phenolic compounds are recognised by the VirA protein, a transmembrane protein encoded in the virA gene on the Ti plasmid. Sugars are recognised by the chvE protein, a chromosomal gene-encoded protein located in the periplasmic space.
- Gelvin SB (March 2003). “Agrobacterium-mediated plant transformation: the biology behind the “gene-jockeying” tool”. Microbiology and Molecular Biology Reviews. 67 (1): 16–37, table of contents. doi:10.1128/mmbr.67.1.16-37.2003. PMC 150518. PMID 12626681.
At least 25 vir genes on the Ti plasmid are necessary for tumor induction[citation needed]. In addition to their perception role, virA and chvE induce other vir genes. The VirA protein has autokinase activity: it phosphorylates itself on a histidine residue. Then the VirA protein phosphorylates the VirG protein on its aspartate residue. The virG protein is a cytoplasmic protein produced from the virG Ti plasmid gene. It is a transcription factor, inducing the transcription of the vir operons. The ChvE protein regulates the second mechanism of the vir genes’ activation. It increases VirA protein sensitivity to phenolic compounds.
- Gelvin SB (March 2003). “Agrobacterium-mediated plant transformation: the biology behind the “gene-jockeying” tool”. Microbiology and Molecular Biology Reviews. 67 (1): 16–37, table of contents. doi:10.1128/mmbr.67.1.16-37.2003. PMC 150518. PMID 12626681.
Attachment is a two-step process. Following an initial weak and reversible attachment, the bacteria synthesize cellulose fibrils that anchor them to the wounded plant cell to which they were attracted. Four main genes are involved in this process: chvA, chvB, pscA, and att. The products of the first three genes apparently are involved in the actual synthesis of the cellulose fibrils. These fibrils also anchor the bacteria to each other, helping to form a microcolony.[citation needed]
VirC, the most important virulent protein, is a necessary step in the recombination of illegitimate recolonization. It selects the section of the DNA in the host plant that will be replaced and it cuts into this strand of DNA.[citation needed]
After production of cellulose fibrils, a calcium-dependent outer membrane protein called rhicadhesin is produced, which also aids in sticking the bacteria to the cell wall. Homologues of this protein can be found in other rhizobia. Currently, there are several reports on standardisation of protocol for the Agrobacterium-mediated transformation. The effect of different parameters such as infection time, acetosyringone, DTT, and cysteine have been studied in soybean (Glycine max).
- Barate PL, Kumar RR, Waghmare SG, Pawar KR, Tabe RH (2018). “Effect of different parameters on Agrobacterium mediated transformation in Glycine max”. International Journal of Advanced Biological Research. 8 (1): 99–105.
Possible plant compounds that initiate Agrobacterium to infect plant cells:
- Acetosyringone and other phenolic compounds
- alpha-Hydroxyacetosyringone
- Catechol
- Ferulic acid
- Gallic acid
- p-Hydroxybenzoic acid
- Protocatechuic acid
- Pyrogallic acid
- Resorcylic acid
- Sinapinic acid
- Syringic acid
- Vanillin
Formation of the T-pilus
To transfer the T-DNA into the plant cell, A. tumefaciens uses a type IV secretion mechanism, involving the production of a T-pilus. When acetosyringone and other substances are detected, a signal transduction event activates the expression of 11 genes within the VirB operon which are responsible for the formation of the T-pilus.
The pro-pilin is formed first. This is a polypeptide of 121 amino acids which requires processing by the removal of 47 residues to form a T-pilus subunit. The subunit was though to be circularized by the formation of a peptide bond between the two ends of the polypeptide. However, high-resolution structure of the T-pilus revealed no cyclization of the pilin, with the overall organization of the pilin subunits being highly similar to those of other conjugative pili, such as F-pilus.
- Beltran, Leticia C.; Cvirkaite-Krupovic, Virginija; Miller, Jessalyn; Wang, Fengbin; Kreutzberger, Mark A. B.; Patkowski, Jonasz B.; Costa, Tiago R. D.; Schouten, Stefan; Levental, Ilya; Conticello, Vincent P.; Egelman, Edward H.; Krupovic, Mart (2023-02-07). “Archaeal DNA-import apparatus is homologous to bacterial conjugation machinery”. Nature Communications. 14 (1): 666. doi:10.1038/s41467-023-36349-8. ISSN 2041-1723. PMC 9905601. PMID 36750723.
Products of the other VirB genes are used to transfer the subunits across the plasma membrane. Yeast two-hybrid studies provide evidence that VirB6, VirB7, VirB8, VirB9 and VirB10 may all encode components of the transporter. An ATPase for the active transport of the subunits would also be required.
Transfer of T-DNA into the plant cell

B: Agrobacterium genome
C: Ti Plasmid: a: T-DNA, b: Vir genes, c: Replication origin, d: Opines catabolism genes
D: Plant cell
E: Mitochondria
F: Chloroplast
G: Nucleus
The T-DNA must be cut out of the circular plasmid. This is typically done by the Vir genes within the helper plasmid. A VirD1/D2 complex nicks the DNA at the left and right border sequences. The VirD2 protein is covalently attached to the 5′ end. VirD2 contains a motif that leads to the nucleoprotein complex being targeted to the type IV secretion system (T4SS). The structure of the T-pilus showed that the central channel of the pilus is too narrow to allow the transfer of the folded VirD2, suggesting that VirD2 must be partially unfolded during the conjugation process.
- Beltran, Leticia C.; Cvirkaite-Krupovic, Virginija; Miller, Jessalyn; Wang, Fengbin; Kreutzberger, Mark A. B.; Patkowski, Jonasz B.; Costa, Tiago R. D.; Schouten, Stefan; Levental, Ilya; Conticello, Vincent P.; Egelman, Edward H.; Krupovic, Mart (2023-02-07). “Archaeal DNA-import apparatus is homologous to bacterial conjugation machinery”. Nature Communications. 14 (1): 666. doi:10.1038/s41467-023-36349-8. ISSN 2041-1723. PMC 9905601. PMID 36750723.
- https://goldbio.com/articles/article/a-guide-to-agrobacterium-t-dna-binary-vectors#_Toc61524553
In the cytoplasm of the recipient cell, the T-DNA complex becomes coated with VirE2 proteins, which are exported through the T4SS independently from the T-DNA complex. Nuclear localization signals, or NLSs, located on the VirE2 and VirD2, are recognised by the importin alpha protein, which then associates with importin beta and the nuclear pore complex to transfer the T-DNA into the nucleus. VIP1 also appears to be an important protein in the process, possibly acting as an adapter to bring the VirE2 to the importin. Once inside the nucleus, VIP2 may target the T-DNA to areas of chromatin that are being actively transcribed, so that the T-DNA can integrate into the host genome.
Genes in the T-DNA
Hormones
To cause gall formation, the T-DNA encodes genes for the production of auxin or indole-3-acetic acid via the IAM pathway. This biosynthetic pathway is not used in many plants for the production of auxin, so it means the plant has no molecular means of regulating it and auxin will be produced constitutively. Genes for the production of cytokinins are also expressed. This stimulates cell proliferation and gall formation.
Opines
The T-DNA contains genes for encoding enzymes that cause the plant to create specialized amino acid derivatives which the bacteria can metabolize, called opines. Opines are a class of chemicals that serve as a source of nitrogen for A. tumefaciens, but not for most other organisms. The specific type of opine produced by A. tumefaciens C58 infected plants is nopaline (Escobar et al., 2003).
- Zupan J, Muth TR, Draper O, Zambryski P (July 2000). “The transfer of DNA from agrobacterium tumefaciens into plants: a feast of fundamental insights”. The Plant Journal. 23 (1): 11–28. doi:10.1046/j.1365-313x.2000.00808.x. PMID 10929098.
Two nopaline type Ti plasmids, pTi-SAKURA and pTiC58, were fully sequenced. “A. fabrum” C58, the first fully sequenced pathovar, was first isolated from a cherry tree crown gall. The genome was simultaneously sequenced by Goodner et al. and Wood et al. in 2001. The genome of A. tumefaciens C58 consists of a circular chromosome, two plasmids, and a linear chromosome. The presence of a covalently bonded circular chromosome is common to Bacteria, with few exceptions. However, the presence of both a single circular chromosome and single linear chromosome is unique to a group in this genus. The two plasmids are pTiC58, responsible for the processes involved in virulence, and pAtC58,[2] once dubbed the “cryptic” plasmid.
- Goodner B, Hinkle G, Gattung S, Miller N, Blanchard M, Qurollo B, et al. (December 2001). “Genome sequence of the plant pathogen and biotechnology agent Agrobacterium tumefaciens C58”. Science. 294 (5550): 2323–8. Bibcode:2001Sci…294.2323G. doi:10.1126/science.1066803. PMID 11743194. S2CID 86255214.
- Wood DW, Setubal JC, Kaul R, Monks DE, Kitajima JP, Okura VK, et al. (December 2001). “The genome of the natural genetic engineer Agrobacterium tumefaciens C58”. Science. 294 (5550): 2317–23. Bibcode:2001Sci…294.2317W. CiteSeerX 10.1.1.7.9501. doi:10.1126/science.1066804. PMID 11743193. S2CID 2761564.
The pAtC58 plasmid has been shown to be involved in the metabolism of opines and to conjugate with other bacteria in the absence of the pTiC58 plasmid. If the Ti plasmid is removed, the tumor growth that is the means of classifying this species of bacteria does not occur.
- Vaudequin-Dransart V, Petit A, Chilton WS, Dessaux Y (1998). “The cryptic plasmid of Agrobacterium tumefaciens cointegrates with the Ti plasmid and cooperates for opine degradation”. Molecular Plant-Microbe Interactions. 11 (7): 583–591. doi:10.1094/mpmi.1998.11.7.583.
Biotechnological uses
The Asilomar Conference (Berg et al. 1975) established widespread agreement that recombinant techniques were insufficiently understood and needed to be tightly controlled. The DNA transmission capabilities of Agrobacterium have been vastly explored in biotechnology as a means of inserting foreign genes into plants. Shortly after Asilomar, Marc Van Montagu and Jeff Schell, (University of Ghent and Plant Genetic Systems, Belgium) discovered the gene transfer mechanism between Agrobacterium and plants, which resulted in the development of methods to alter the bacterium into an efficient delivery system for genetic engineering in plants. The plasmid T-DNA that is transferred to the plant is an ideal vehicle for genetic engineering. This is done by cloning a desired gene sequence into T-DNA binary vectors that will be used to deliver a sequence of interest into eukaryotic cells. Soon it was widely agreed that Asilomar like protections were needed in plant technologies as well. This process has been performed using firefly luciferase gene to produce glowing plants.[citation needed] This luminescence has been a useful device in the study of plant chloroplast function and as a reporter gene. It is also possible to transform Arabidopsis thaliana by dipping flowers into a broth of Agrobacterium: the seed produced will be transgenic. Under laboratory conditions, the T-DNA has also been transferred to human cells, demonstrating the diversity of insertion application.
- Genetically Modified Pest-Protected Plants: Science and Regulation. Washington, D.C.: National Academies Press. 2000. pp. xxiii+263. ISBN 978-0-309-06930-4. OCLC 894124744.
- Schell J, Van Montagu M (1977). “The Ti-plasmid of Agrobacterium tumefaciens, a natural vector for the introduction of nif genes in plants?”. Basic Life Sciences. 9: 159–79. doi:10.1007/978-1-4684-0880-5_12. ISBN 978-1-4684-0882-9. PMID 336023.
- Zambryski P, Joos H, Genetello C, Leemans J, Montagu MV, Schell J (1983). “Ti plasmid vector for the introduction of DNA into plant cells without alteration of their normal regeneration capacity”. The EMBO Journal. 2 (12): 2143–50. doi:10.1002/j.1460-2075.1983.tb01715.x. PMC 555426. PMID 16453482.
- Root M (1988). “Glow in the dark biotechnology”. BioScience. 38 (11): 745–747. doi:10.2307/1310781. JSTOR 1310781.
- Kunik T, Tzfira T, Kapulnik Y, Gafni Y, Dingwall C, Citovsky V (February 2001). “Genetic transformation of HeLa cells by Agrobacterium”. Proceedings of the National Academy of Sciences of the United States of America. 98 (4): 1871–6. Bibcode:2001PNAS…98.1871K. doi:10.1073/pnas.041327598. PMC 29349. PMID 11172043.
The mechanism by which Agrobacterium inserts materials into the host cell is by a type IV secretion system which is very similar to mechanisms used by pathogens to insert materials (usually proteins) into human cells by type III secretion. It also employs a type of signaling conserved in many Gram-negative bacteria called quorum sensing[citation needed]. This makes Agrobacterium an important topic of medical research, as well[citation needed].
Natural genetic transformation
Natural genetic transformation in bacteria is a sexual process involving the transfer of DNA from one cell to another through the intervening medium, and the integration of the donor sequence into the recipient genome by homologous recombination. A. tumefaciens can undergo natural transformation in soil without any specific physical or chemical treatment.
- Demanèche S, Kay E, Gourbière F, Simonet P (June 2001). “Natural transformation of Pseudomonas fluorescens and Agrobacterium tumefaciens in soil”. Applied and Environmental Microbiology. 67 (6): 2617–21. Bibcode:2001ApEnM..67.2617D. doi:10.1128/AEM.67.6.2617-2621.2001. PMC 92915. PMID 11375171.
Disease cycle
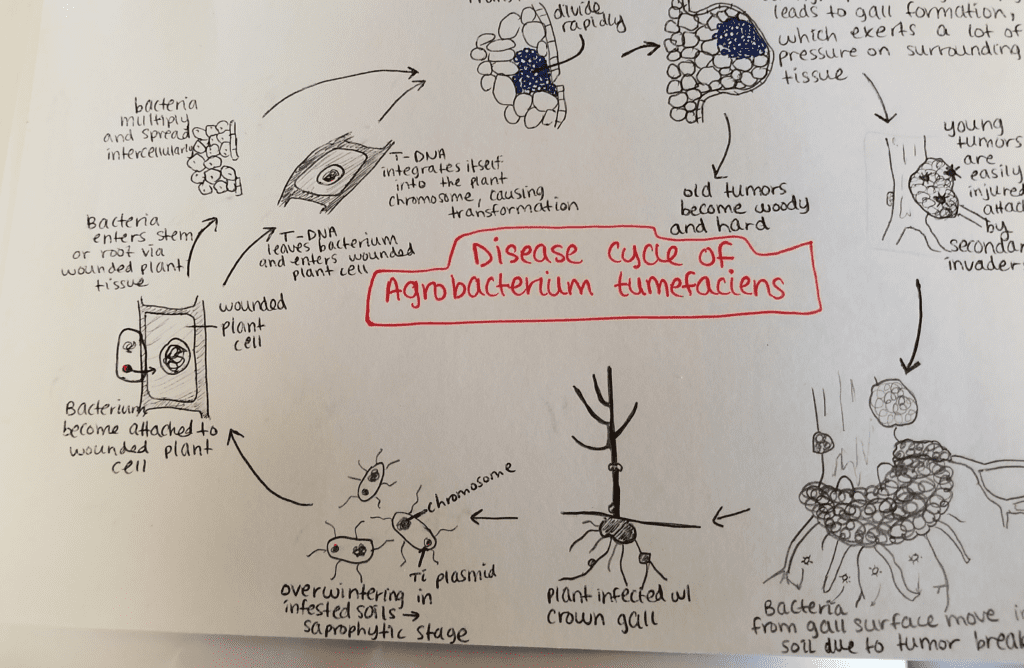
Agrobacterium tumefaciens overwinters in infested soils. Agrobacterium species live predominantly saprophytic lifestyles, so its common even for plant-parasitic species of this genus to survive in the soil for lengthy periods of time, even without host plant presence. When there is a host plant present, however, the bacteria enter the plant tissue via recent wounds or natural openings of roots or stems near the ground. These wounds may be caused by cultural practices, grafting, insects, etc. Once the bacteria have entered the plant, they occur intercellularly and stimulate surrounding tissue to proliferate due to cell transformation. Agrobacterium performs this control by inserting the plasmid T-DNA into the plant’s genome. See above for more details about the process of plasmid DNA insertion into the host genome. Excess growth of the plant tissue leads to gall formation on the stem and roots. These tumors exert significant pressure on the surrounding plant tissue, which causes this tissue to become crushed and/or distorted. The crushed vessels lead to reduced water flow in the xylem. Young tumors are soft and therefore vulnerable to secondary invasion by insects and saprophytic microorganisms. This secondary invasion causes the breakdown of the peripheral cell layers as well as tumor discoloration due to decay. Breakdown of the soft tissue leads to release of the Agrobacterium tumefaciens into the soil allowing it to restart the disease process with a new host plant.
- Schroth MN, Weinhold AR, Mccain AH (March 1971). “Biology and Control of Agrobacterium tumefaciens”. Hilgardia. 40 (15): 537–552. doi:10.3733/hilg.v40n15p537.
- Agrios GN (2005). Plant pathology (5th ed.). Amsterdam: Elsevier Academic Press. ISBN 9780120445653. OCLC 55488155.
Disease management
Crown gall disease caused by Agrobacterium tumefaciens can be controlled by using various methods. The best way to control this disease is to take preventative measures, such as sterilizing pruning tools so as to avoid infecting new plants. Performing mandatory inspections of nursery stock and rejecting infected plants as well as not planting susceptible plants in infected fields are also valuable practices. Avoiding wounding the crowns/roots of the plants during cultivation is important for preventing disease. In horticultural techniques in which multiple plants are joined to grow as one, such as budding and grafting these techniques lead to plant wounds. Wounds are the primary location of bacterial entry into the host plant. Therefore, it is advisable to perform these techniques during times of the year when Agrobacteria are not active. Control of root-chewing insects is also helpful to reduce levels of infection, since these insects cause wounds (aka bacterial entryways) in the plant roots. It is recommended that infected plant material be burned rather than placed in a compost pile due to the bacteria’s ability to live in the soil for many years.
- Agrios GN (2005). Plant pathology (5th ed.). Amsterdam: Elsevier Academic Press. ISBN 9780120445653. OCLC 55488155.
- “Grafting and Budding Nursery Crop Plants”. content.ces.ncsu.edu. NC State Extension Publications. Retrieved 2017-12-02.
- “Crown gall : Yard and Garden: Garden”. University of Minnesota Extension. Archived from the original on 2017-10-16. Retrieved 2017-10-15.
Biological control methods are also utilized in managing this disease. During the 1970s and 1980s, a common practice for treating germinated seeds, seedlings, and rootstock was to soak them in a suspension of K84. K84 is composed of A. radiobacter, which is a species related to A. tumefaciens but is not pathogenic. K84 produces a bacteriocin (agrocin 84) which is an antibiotic specific against related bacteria, including A. tumefaciens. This method, which was successful at controlling the disease on a commercial scale, had the risk of K84 transferring its resistance gene to the pathogenic Agrobacteria. Thus, in the 1990s, the use of a genetically engineering strain of K84, known as K-1026, was created. This strain is just as successful in controlling crown gall as K84 without the caveat of resistance gene transfer.
- Ryder MH, Jones DA (1991-10-01). “Biological Control of Crown Gall Using Using Agrobacterium Strains K84 and K1026”. Functional Plant Biology. 18 (5): 571–579. doi:10.1071/pp9910571.
Environment
Host, environment, and pathogen are extremely important concepts in regards to plant pathology. Agrobacteria have the widest host range of any plant pathogen, so the main factor to take into consideration in the case of crown gall is environment. There are various conditions and factors that make for a conducive environment for A. tumefaciens when infecting its various hosts. The bacterium can’t penetrate the host plant without an entry point such as a wound. Factors leading to wounds in plants include cultural practices, grafting, freezing injury, growth cracks, soil insects, and other animals in the environment causing damage to the plant. Consequently, in exceptionally harsh winters, it is common to have an increased incidence of crown gall due to the weather-related damage. Along with this, there are methods of mediating infection of the host plant. For example, nematodes can act as a vector to introduce Agrobacterium into plant roots. More specifically, the root parasitic nematodes damage the plant cell, creating a wound for the bacteria to enter through. Finally, temperature is a factor when considering A. tumefaciens infection. The optimal temperature for crown gall formation due to this bacterium is 22 °C (72 °F) because of the thermosensitivity of T-DNA transfer. Tumor formation is significantly reduced at higher temperature conditions.
- “Bacterial Crown Gall of Fruit Crops | Ohioline”. ohioline.osu.edu. Retrieved 2017-10-20.
- “Crown Gall – A Growing Concern in Vineyards”. extension.psu.edu. Archived from the original on 2017-10-20. Retrieved 2017-10-20.
- Karimi M, Van Montagu M, Gheysen G (November 2000). “Nematodes as vectors to introduce Agrobacterium into plant roots”. Molecular Plant Pathology. 1 (6): 383–7. doi:10.1046/j.1364-3703.2000.00043.x. PMID 20572986. S2CID 35932276.
- Dillen W, De Clereq J, Kapila J, Van Montagu ZM, Angenon G (1997-12-01). “The effect of temperature on Agrobacterium tumefaciens-mediated gene transfer to plants”. The Plant Journal. 12 (6): 1459–1463. doi:10.1046/j.1365-313x.1997.12061459.x.
See also
Note
- This is contested: Velazquez E, Flores-Felix JD, Sanchez-Juanes F, Igual JM, Peix A (2020). “Strain ATCC 4720T is the authentic type strain of Agrobacterium tumefaciens, which is not a later heterotypic synonym of Agrobacterium radiobacter“. Int J Syst Evol Microbiol. 70 (9): 5172–5176. doi:10.1099/ijsem.0.004443. PMID 32915125.
- “At plasmid” when talking about related plasmids
Further reading
- Dickinson M (2003). Molecular Plant Pathology. BIOS Scientific Publishers.
- Lai EM, Kado CI (August 2000). “The T-pilus of Agrobacterium tumefaciens“. Trends in Microbiology. 8 (8): 361–9. doi:10.1016/s0966-842x(00)01802-3. PMID 10920395.
- Ward DV, Zupan JR, Zambryski PC (January 2002). “Agrobacterium VirE2 gets the VIP1 treatment in plant nuclear import”. Trends in Plant Science. 7 (1): 1–3. doi:10.1016/s1360-1385(01)02175-6. PMID 11804814.
- Webster J, Thomson J (1988). “Genetic Analysis of an Agrobacterium tumefaciens strain producing an agrocin active against biotype 3 Pathogen”. Molecular and General Genetics. 214 (1): 142–147. doi:10.1007/BF00340192. S2CID 180063.
External links
- Media related to Agrobacterium tumefaciens at Wikimedia Commons
- “Agrobacterium fabrum” C58 Genome Page — as sequenced by Cereon Genomics/University of Richmond
- Gram-negative bacteria
- Bacterial plant pathogens and diseases
- Bacterial grape diseases
- Bacteria described in 1907
- Rhizobiaceae
Fungal diseases
Fungal diseases | |
---|---|
Anthracnose | Piggotia coryli = Monostichella coryli = Gloeosporium coryli = Labrella coryli |
Armillaria root disease | Armillaria spp. |
Borro sec | Cryptosporiopsis tarraconensis |
Cytospora canker | Cytospora spp. |
Eastern filbert blight | Anisogramma anomala |
Kernel molds | Mycosphaerella punctiformis [teleomorph] Ramularia sp. [anamorph] Phomopsis spp. Septoria ostryae |
Kernel spot | Nematospora coryli |
Leaf blister | Taphrina coryli |
Leaf spots | Anguillosporella vermiformis Asteroma coryli Cercospora corylina Cercospora coryli Mamianiella coryli Monochaetia coryli Mycosphaerella punctiformis [teleomorph] Ramularia sp. [anamorph] Phyllosticta coryli Ramularia coryli Septoria ostryae Sphaceloma coryli |
Nectria canker | Nectria ditissima |
Texas root rot | Phymatotrichopsis omnivora |
Powdery mildew | Microsphaera coryli Microsphaera ellisii Microsphaera hommae Microsphaera verruculosa Phyllactinia guttata = Phyllactinia suffulta |
Rust | Pucciniastrum coryli |
Viral diseases
Viral diseases | |
---|---|
Hazelnut mosaic | genus Ilarvirus, Apple mosaic virus (ApMV) genus Ilarvirus, Prunus necrotic ringspot virus (PNRSV) genus Ilarvirus, Tulare apple mosaic virus (TAMV) |
Phytoplasmal and spiroplasmal diseases
Phytoplasmal and spiroplasmal diseases | |
---|---|
Filbert Stunt | unknown, suspect a phytoplasma |
Hazelnut Yellows | phytoplasma |
Miscellaneous diseases and disorders
Miscellaneous diseases and disorders | |
---|---|
Blanks | empty nut shells, cause unknown |
Brown Stain | brown liquefied portions of shell and kernel, cause unknown |
Catkin Blast | deformed catkins, cause unknown |
Sun Scald | high temperature |
Wet Feet | saturated soil conditions for extended periods. |